The content of this book is licensed under a Creative Commons Attribution-NonCommercial-NoDerivs 4.0 Unported license. To view the terms and conditions of this license, visit https://creativecommons.org/licenses/by-nc-nd/4.0/
NCBI Bookshelf. A service of the National Library of Medicine, National Institutes of Health.
Varki A, Cummings RD, Esko JD, et al., editors. Essentials of Glycobiology [Internet]. 4th edition. Cold Spring Harbor (NY): Cold Spring Harbor Laboratory Press; 2022. doi: 10.1101/glycobiology.4e.50
This chapter surveys techniques for structural characterization of glycans, including composition, linkage, and attachment to aglycones. It covers detection of specific glycan sequences on glycoproteins and cellular surfaces and methods for characterizing structures in three dimensions. The techniques described range from classical chemical detection and characterization of isolated glycan products to sensitive fluorescence methods used in combination with glycan-binding proteins and microscopy on cells and tissues. In addition, nuclear magnetic resonance spectroscopy (NMR) and mass spectrometry (MS), approaches that allow more detailed structural characterization, are discussed.
BACKGROUND
The primary structure of a glycan is defined by the type and order of monosaccharide residues, by the configuration and position of glycosidic linkages, and by the nature and location of the nonglycan entity to which it is attached (the aglycone); see Chapters 2 and 3. For glycoproteins, different glycans can be attached to different sites in the protein, and these glycans can vary when that glycoprotein is made in different cell types or at different stages of development. Moreover, it is often three-dimensional (3D) features, or particular surface distributions of glycans, that are recognized by glycan-binding proteins. Characterization of these diverse structural features requires an array of different methods, with the choice of methodology depending very much on the problem.
For a typical mammalian glycoprotein, the aim is often to identify the correct glycan structure from a range of known or predictable candidate structures, and a limited amount of structural data may suffice (Chapter 51). For glycans from bacteria or less well-characterized organisms, it is hard to make predictions, and therefore, a more complete data set may be required. The choice of methodology also depends on the amount and purity of material available, as well as the context in which data must be collected (e.g., tissue vs. isolated glycoprotein). If quantities are not limiting, the complete primary structure and even the tertiary (3D) structure may be determined. The need to respond to diverse circumstances and to understand the complexities of glycan structure has driven the development of many of the methods described in the following sections. The level of glycan structural characterization required to address the specific biological question that is being investigated can also direct experimental approaches.
DETECTION OF GLYCANS
Methods for glycan detection in glycoconjugates include direct chemical reactions with the constituent monosaccharides, metabolic labeling with either radioactive or chemically reactive monosaccharides, and detection with specific glycan-recognizing proteins (including lectins and antibodies) (Chapter 48). A general method for detecting the presence of glycans on proteins involves periodate oxidation of their hydroxyl groups followed by Schiff base formation with amine- or hydrazide-based probes (Chapter 2). This chemical modification, also known as the periodic acid–Schiff (PAS) reaction, can identify glycoproteins in gels. Commercially available kits allow detection of 5–10 ng of glycoprotein, using the periodate reaction with subsequent amplification by means of biotin-hydrazide/streptavidin-alkaline phosphatase or fluorescence-based detection. Lectin overlay of a blot of a sodium dodecyl sulfate polyacrylamide gel electrophoresis (SDS-PAGE) gel can detect the presence of specific glycans with comparable sensitivity and greater specificity. For example, the agglutinin from Sambucus nigra (SNA) binds to glycans that terminate with α2-6 sialic acid (Sia). Lectins (Chapters 31–36) recognizing terminal fucose (Fuc), galactose (Gal), N-acetylgalactosamine (GalNAc), and N-acetylglucosamine (GlcNAc) are also commercially available.
Metabolic labeling of glycoconjugates with radioactive sugars is another powerful tool for determining the composition of glycans. Cells incubated in media containing 3H- or 14C-labeled monosaccharides will incorporate the label into the glycans of glycoconjugates. Radiolabeled glycans can be detected following gel electrophoresis (SDS-PAGE) or thin-layer chromatography (TLC) by autoradiography or fluorography. They can also be released and studied in detail by various methods. The use of fluorescent probes and labels has enabled a reduction in the use of radioisotopes in applications in which the detection and quantitative determination of glycans, as opposed to glycosylation pathway information, is the primary objective. Fluorescent labels for sensitive detection of glycans after liquid chromatography (LC) include readily available 2-amino benzoic acid (2-AA) and 2-aminobenzamide (2-AB), which may be attached by reductive amination to the reducing sugar exposed on release of glycans from an aglycone.
Metabolic labeling can also be performed with synthetic monosaccharides that are modified with chemically reactive groups. For example, the azido monosaccharide N-azidoacetylmannosamine (ManNAz) is converted by cells to N-azidoacetyl Sia (SiaNAz), which is incorporated into sialylated glycans in place of a natural Sia. The azido group can then be selectively reacted with phosphine or alkyne reagents (Chapter 53) that introduce a fluorescent dye or an affinity probe such as biotin, thereby enabling detection of Sia in cells. Azido analogs of GalNAc and GlcNAc can be used to label O-GalNAc (Chapter 10) or O-GlcNAc glycans (Chapters 13 and 19), respectively. Use of fluorescent labels can also be coupled with confocal microscopy to give important insights into the location of glycans in cells and tissues. However, such chemical modifications may change the biosynthesis and/or biology of glycans, creating some uncertainty about the observed results, and no method has completely supplanted radioactive metabolic labeling for pulse-chase studies of naturally occurring glycans.
Glycoproteins
A glycosylated protein typically presents one or more diffuse bands during gel electrophoresis, resulting from heterogeneity of the attached glycans. Even when visualized by protein staining reagents, this phenomenon is often the first indication of the presence of glycans. Some high-molecular-weight glycoconjugates, such as mucins and proteoglycans, do not enter ordinary gels or, if they do, they migrate as heterogeneous smears. Agarose gels or combination polyacrylamide-agarose gels may be useful in this situation. Several analytical options are available to investigate the presence of glycans further (e.g., the PAS stain described above). Treatment of glycoproteins with endoglycosidases (e.g., peptide-N-glycosidase F [PNGase F], endoglycosidase F2 [Endo F2], endoglycosidase H [Endo H]) is another option; see Table 50.1 and Figure 50.1. If this results in a mobility change of one or more of the bands on the gel, the presence of N-glycans is indicated. O-glycanase (endo-α-N-acetylgalactosaminidase; Table 50.1) can be used for the specific identification of O-glycans. However, depending on the structure of the O-glycan, pretreatment with other enzymes to expose the disaccharide core is sometimes required. Removal of individual sugars by exoglycosidases such as sialidase or β-galactosidase (Figure 50.1) may also result in a mobility change if a sufficient number of residues is removed. Some glycans cannot be altered by these treatments as they are resistant to the enzymes used. Resistance can result from modifications to glycan hydroxyl groups (e.g., sulfation, acetylation, or phosphorylation; Chapter 2), glycosidic linkages that are not recognized by the enzymes, or steric inaccessibility of the glycan. Complete removal of N- and O-glycans can be achieved by chemical treatments (e.g., hydrazinolysis or β-elimination), but peptide damage usually precludes further analysis by gel electrophoresis. Partial degradation (e.g., by loss of O-acetylation) of the glycan may also occur.
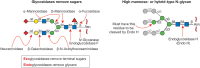
FIGURE 50.1.
Glycosidases used for structural analysis. (Left) A biantennary N-glycan is shown with exoglycosidases that remove each monosaccharide sequentially. Exoglycosidases act only on terminal sugars. Also shown are endoglycosidases that digest in the core region (more...)
TABLE 50.1.
Table of enzymes for glycan analysis
Proteoglycans
Proteoglycans (Chapter 17) may be separated by agarose gel electrophoresis and by ion-exchange chromatography, which separates on the basis of charge conferred by sulfate groups. Treatment of proteoglycans with GAG lyases (Table 50.1) will produce a shift in mobility on a gel, condensing the proteoglycan smear into discrete bands. After removal of much of the glycan portion, antibodies that recognize the remaining “stub” glycans may be used in western analysis.
Glycolipids
Typically, the analysis of glycolipid glycans by NMR or MS is preceded by their chromatographic purification. Mixtures of glycolipids can be fractionated by TLC and TLC plates stained with glycan-reactive reagents, allowing detection of many individual glycolipids. Using different reagents, it is possible to recognize gangliosides (e.g., resorcinol-HCl detects Sia) or neutral monosaccharides (e.g., orcinol-sulfuric acid detects all monosaccharides) in TLC bands. Reagents are also available for detection of sulfate and phosphate groups on glycolipids. Some pre-purification of the crude extract is usually required (e.g., Folch partitioning and ion-exchange chromatography). These procedures separate nonpolar or nonionic lipids from polar lipids (e.g., glycosphingolipids; Chapter 11) and those that contain charged groups (i.e., gangliosides, phospholipids, and sulfatides). It is also common practice to deduce the presence of specific sugars by evaluating the shifts produced in the migration position of a band following a chemical or enzymatic treatment. Glycolipids on TLC plates can also be detected by monoclonal antibodies, lectins, or even intact microorganisms expressing glycan-specific receptors (Chapter 48). Detailed structural features may be identified by running the TLC in a second dimension following a specific treatment. On a larger scale, glycolipids are separated using column chromatography or by high-performance TLC on silica plates.
GPI Anchors
GPI-anchored proteins (Chapter 12), with their lipid, protein, and glycan constituents, have unique physicochemical properties that can be exploited for their detection. The nonionic detergent Triton X-114 at low temperature (4°C) extracts soluble and integral membrane proteins, as well as GPI-anchored proteins. When the solution is warmed, two phases separate, and GPI-anchored and other amphiphilic proteins remain associated with the detergent-enriched phase. GPI-specific phospholipases can be used to cleave GPI anchors for further characterization. Successful cleavage by GPI-specific phospholipases can be assessed using SDS-PAGE, because removal of the GPI anchor causes a shift in molecular mass. This is a common diagnostic method for identifying the presence of a GPI anchor on a protein of interest. Another method is to treat the GPI-anchored protein with nitrous acid, which cleaves the unsubstituted glucosamine residue that links the glycan to the phosphatidylinositol.
Plant and Bacterial Polysaccharides
This class of glycans contains many structures, including homopolysaccharides and heteropolysaccharides, neutral and ionic polysaccharides, and linear and branched structures, with widespread molecular sizes ranging from a few monosaccharide units to thousands (Chapters 3, 21, and 22). Most of these polymers are insoluble in aqueous solutions, occurring as complex and sometimes crystalline aggregates. But many can be extracted with water, salts, chaotropic agents, or detergents and isolated by precipitation with alcohols. Detection is based on refractive index (RI) or colorimetric reactions, because sample quantity is not usually a limitation.
RELEASE AND SEPARATION OF GLYCANS
Once the presence and general type of glycan has been established, the next challenge is to release specific types of glycans and separate different classes in sufficient quantities for structural characterization.
Release of Glycans from Glycoconjugates
When glycans are released before structural analysis, it is best to use a quantitative release procedure that neither destroys nor alters the glycan. Ideally, information regarding the type of linkage between the glycan and its liberated protein or lipid should be retained, although this is not always possible. Glycolipids can often be isolated and characterized by MS and/or NMR without the need for release of glycans, but, if necessary, enzymatic methods can be used, or for glycosphingolipids, ozonolysis will separate lipid from glycan. Complex, hybrid and oligomannose N-glycans can be released from glycoproteins with N-glycosidases such as PNGase F or PNGase A (Table 50.1). Endo H may be used for the selective release of high-mannose and hybrid N-glycans, but complex N-glycans are resistant (Table 50.1; Figure 50.1).
Chemical approaches suitable for release of glycans from a protein include hydrazinolysis, which releases N-glycans and/or O-glycans, depending on experimental conditions. Strong base treatment can, under carefully controlled conditions, release only O-glycans in a process called β-elimination; it is generally accompanied by reduction with borohydride to give an alditol. More recently methods have been developed in which base treatment is accompanied by derivatization with pyrazolone, which acts as a UV-absorbing label during chromatographic separation. However, all of the above chemical methods can result in partial or complete loss of labile modifications of the glycan, such as O-acetylation or sulfation, and degradation of the protein.
Separation of Glycans
Glycans released from a glycoconjugate usually form a complex mixture. Even when only one glycosylation site in the protein is occupied, individual molecules can bear different glycan species generating multiple glycoforms. The high-throughput analysis of these glycan mixtures using glycomics technologies is described in Chapter 51.
Preparation of glycan samples for structural analysis will rely on separation techniques. Chromatographic separations commonly used to isolate pure glycans, or at least glycan mixtures of reduced complexity, include size exclusion chromatography (SEC), strong or weak anion exchange chromatography (SAX or WAX), and some forms of reverse-phase high-pressure liquid chromatography (HPLC). Electrophoretic methods include capillary electrophoresis (CE) and fluorophore-assisted carbohydrate electrophoresis (FACE).
HPLC-based separation is feasible, even for large amounts (>10 mg), if the mixture contains fewer than approximately 50 glycan species. Individual fractions can be recovered for further structural analysis by MS or NMR. Once liberated from their glycoconjugates, glycans with free-reducing termini (Chapter 2) can be chemically labeled with fluorescent tags, such as 2-amino benzoic acid (2-AA) and 2-aminobenzamide (2-AB) or 8-aminonaphthalene-1,3,6-trisulfonic acid (ANTS), providing detection sensitivity that rivals the sensitivity achieved with radiolabels. Advantages of this method include more facile purification of the labeled glycans and a wider variety of options for chromatographic separations and structural analysis techniques. Nonlabeled glycans may be detected by (relatively insensitive) RI methods, or by more sensitive pulsed amperometric detection (PAD).
If a label is introduced at the reducing end, it is possible to use sequential exoglycosidase treatments (Table 50.1; Figure 50.1) and look for shifts of the oligosaccharide in a chromatographic system (e.g., paper chromatography, HPLC, TLC) as an indication of susceptibility to the enzyme. Comparison with known standards treated in the same manner allows tentative glycan identification. However, well-characterized standards of known structure are difficult to obtain in a pure form and there are nearly always “contaminating” peaks in a chromatogram that cannot be readily identified. It is very important to note that separation profiling should not be confused with actual structural analysis, because coelution with a standard could occur with a variety of different structures.
MONOSACCHARIDE COMPOSITION ANALYSIS
Some qualitative information on monosaccharide composition of a glycan may be derived from the procedures described above. However, it is often convenient and informative to determine monosaccharide composition without prior release of glycans. After total hydrolysis of a glycan into its monosaccharide constituents, colorimetric reactions can be used to determine the total amount of hexose, hexuronic acid, or hexosamine in a sample. These approaches only require common reagents and a spectrophotometer, but determination of total glycan content may not always be accurate because of variations in the sensitivities of different linkages to hydrolysis, variations in the degradation of individual saccharides, or a lack of specificity and/or sensitivity in the assays.
Quantitative monosaccharide analysis provides estimated molar ratios of individual monosaccharide components of glycans and may suggest the presence of specific oligosaccharide classes (e.g., N-glycans vs. O-glycans). The analysis involves the following steps: cleavage of all glycosidic linkages (typically by acid hydrolysis), fractionation of the resulting monosaccharides, detection, and quantification. Since the early 1960s, a variety of gas chromatography (GC) methods have been developed to quantify monosaccharides. The most useful involve coupling of GC and MS for linkage and composition information. GC requires volatile samples so the monosaccharides are first chemically modified at their hydroxyl, amide, carboxyl, and aldehyde groups. Reduction of the aldehyde of free monosaccharides followed by acetylation of their hydroxyl groups provides derivatives termed “alditol acetates.” These modified monosaccharides can be readily analyzed by GC-MS and compared with authentic standards. The hydroxyl and amino groups of free monosaccharides generated by glycan hydrolysis can also be converted to trimethylsilyl ethers. These per-O-trimethylsilyl derivatives are widely used for monosaccharide compositional analysis by GC-MS. Incorporation of an optically pure chiral aglycone (e.g., [–]-2-butyl alcohol), in combination with trimethylsilylation, allows the GC separation of D and L isomers and thus determination of the absolute configuration of each monosaccharide.
An alternative to GC-MS is high-pH anion-exchange chromatography with pulsed amperometric detection (HPAEC-PAD), a special type of ion exchange chromatography which does not require monosaccharide derivatization. This technique is especially useful for analyzing acidic sugars such as Sia, which are refractory to GC-MS. A convenient method for quantitating Sia, that does not require expensive equipment, involves tagging with 1,2-diamino-4,5-methylene-dioxybenzene and measuring fluorescence. This method has a detection sensitivity in the femtomole range, and can also pick up many of the naturally occurring modifications of this diverse class of monosaccharides (Chapter 15). Other popular techniques for defining monosaccharide composition are HPLC and high-performance capillary electrophoresis (HPCE). The monosaccharides are usually tagged with a fluorescent derivative for high-sensitivity detection. Tagging with 8-amino-1,3,6-naphthalene trisulfonic acid yields anionic derivatives that can be conveniently analyzed by gel electrophoresis. This is referred to as fluorophore-assisted carbohydrate electrophoresis (FACE).
LINKAGE ANALYSIS
Determination of Linkage Positions
Linkage analysis is a well-established and ingenious approach for determining linkage positions. The principle of this method is to introduce a stable substituent (an ether-linked methyl group) onto each free hydroxyl group of the native glycan. Glycosidic linkages are then cleaved by acid hydrolysis, producing partially methylated monosaccharides with free hydroxyl groups at the positions that were previously involved in a linkage. The partially methylated monosaccharides are ring-opened with a reducing agent (normally borodeuteride) to introduce a new hydroxyl group and, more importantly, a deuterium atom at C-1, which helps identify the reducing end of each monosaccharide. All the free hydroxyl groups are then acetylated resulting in partially methylated alditol acetates (PMAAs) that can be identified by a combination of GC retention times and electron impact (EI)-MS (Figure 50.2). The masses of fragments produced by impact of high-energy electrons on PMAAs identify substitution sites in some cases, but fragmentation patterns of similarly substituted isomeric monosaccharides (e.g., Glc and Gal) can be nearly identical. Thus, definitive identification of monosaccharides requires, in addition to the analysis of the MS pattern, a comparison of GC retention times with those of known standards (e.g., all peracetylated 2,3,4-tri-O-methyl-hexoses produce the same EI-MS spectrum, but peracetylated 2,3,4-tri-O-methyl-galactitol elutes later than peracetylated 2,3,4-tri-O-methyl-glucitol). This type of analysis identifies terminal residues (they are methylated at every position except the hydroxyl group at C-1 and the ring oxygen), indicates how each monosaccharide is substituted, including the occurrence of linkage and branching points, and allows the determination of the ring size (pyranose [p] or furanose [f]) for each monosaccharide. However, linkage analysis provides no information on the nature of substituents or sequence information and cannot reveal α- or β-anomeric configuration.

FIGURE 50.2.
(See following page.) An example of linkage analysis showing a bacterial O-linked branched hexasaccharide with a sequence of Rha1-3Glc1-(Glc1-3GlcNAc1-)2,6Glc1-6GlcNAc. The O-glycan is reductively eliminated from protein before successive steps of permethylation, (more...)
Mass Spectrometry
EI-MS in monosaccharide composition and linkage analyses is covered above. In this section, the other main types of ionization used in MS analysis of glycans—matrix-assisted laser desorption/ionization (MALDI) and electrospray ionization (ESI)—are described. These technologies permit the direct ionization of nonvolatile substances, and are applicable to intact glycoconjugates, as well as fragments thereof. These techniques continue to exploit sample handling strategies and knowledge of glycan fragmentation pathways originally developed for the earlier fast atom bombardment (FAB)-MS. Among the structural features that can be defined by MS methods are (1) degree of heterogeneity and type of glycosylation (e.g., N-glycan vs. O-glycan; oligomannose, hybrid, or complex N-glycans); (2) sites of glycosylation and identity of the protein/lipid carrier; (3) glycan branching; (4) number and lengths of antennae, their composition, and substitution with Fuc, Sia, or other capping groups such as sulfate, phosphate, or acetyl esters; and (5) complete sequences of individual glycans.
In MALDI-MS experiments, the sample is dried on a metal target in the presence of a light-absorbing matrix until matrix crystals containing trapped sample molecules are formed. Ionization of the sample is affected by energy transfer from matrix molecules that have absorbed energy from laser pulses. MALDI-MS is the preeminent technique for screening for molecular ions in complex mixtures, and it is a powerful tool for glycomic profiling (Chapter 51). For ESI-MS, a stream of liquid containing the sample enters the source through a capillary interface, where the sample molecules are stripped of solvent, leaving them as multiply charged species. ESI-MS can be coupled to nano- or capillary-bore LC permitting online LC/ESI-MS analysis. This method is particularly useful when complex mixtures of peptides and glycopeptides are being analyzed (e.g., after proteolytic digestion of a glycoprotein).
In principle, MS provides two types of structural information—the masses of intact molecules (the molecular ions) and the masses of fragment ions. However, in both ESI- and MALDI-MS, the ionization process is usually not sufficiently energetic for fragment ion formation. To overcome this, ESI and MALDI instruments normally have two analyzers in tandem, which allows detection by the second analyzer, of fragment ions produced after molecular ions selected by the first analyzer undergo collisions with an inert gas in a chamber placed between the two analyzers. This is referred to as collision-activated dissociation (CAD) or collision-induced dissociation (CID). MALDI-MS/MS is commonly performed on instruments that have two time-of-flight (TOF) analyzers in tandem (TOF-TOF), whereas the most popular instruments for ESI-MS/MS have either a quadrupole (Q) as the first analyzer and an orthogonal TOF as the second analyzer (QTOF) or a linear ion trap as the first analyzer and an Orbitrap as the second analyzer (LTQ-Orbitrap). Some MS/MS instruments have a single “ion trap” analyzer that traps ions and functions as the collision chamber. Ion traps are relatively inexpensive, but they lack the sensitivity, versatility, and mass range of most tandem instruments. The Fourier transform mass spectrometer (FTMS), a very expensive but very powerful instrument, has extremely high mass accuracy. An alternative to CAD for fragment ion production has been developed for FTMS. This is called electron capture dissociation (ECD) and involves the capture of an electron by the multiply charged molecular ion. A related technique, called electron transfer dissociation (ETD) was subsequently developed for tandem instruments. Both ECD and ETD yield radical cations generated by different fragmentation pathways than ions produced by CAD. ECD and ETD are especially useful for defining glycosylation sites in glycopeptides.
Although underivatized glycans can be analyzed by MALDI- or ESI-MS/MS, superior data can be obtained if the glycans are derivatized before MS analysis. Derivatization methods can be broadly divided into two categories: (1) “tagging” of reducing ends and (2) protection of all functional groups. Commonly used tagging reagents include p-aminobenzoic acid ethyl ester, 2-AP and 2-AB. This type of derivatization facilitates chromatographic purification and enhances useful fragmentation. Protection of hydroxyl groups by permethylation is by far the most important type of full derivatization used in glycan MS (although with accompanying destruction of acetyl esters and other base-labile functional groups during the derivatization process). In MS/MS experiments, permethylated derivatives form abundant fragment ions produced by cleavage at susceptible glycosidic linkages, notably on the reducing side of each HexNAc residue. In N- and O-glycans, this preferred fragmentation unambiguously establishes the antennae sequences. MALDI- and ESI-MS can equally be applied to the characterization of glycolipid and GAG derived glycans. However, in the latter case, because of the size and high degree of sulfation of GAGs, MS analysis is usually performed after hydrolysis of the polymeric chain.
As described above, mass spectrometry measures the mass to charge ratio of glycans during the analytical process. However, as multiple monosaccharides that make up a glycan can have the same mass (e.g., mannose, galactose and glucose) and monosaccharides can be linked together in different ways, a single peak in a glycan mass spectrum can actually be made up of multiple structural isomers. Ion mobility mass spectrometry additionally separates ions based on their size and shape which can resolve glycan structural isomers with the same mass to charge ratio (see Further Reading).
Broadly speaking, the unique strengths of MS can be exploited in two general ways in glycobiology. The first is to obtain detailed characterization of purified individual glycans or mixtures of glycans. In this type of study, it is essential to acquire sufficient rigorous data to define structure unambiguously; many different MS-based experiments will be required, often complemented by NMR, linkage analysis, and profiling of enzyme digests. The second is to pursue glycomics investigations in situations in which it may not be essential to define structures fully, and high-throughput glycomic profiling or mass-mapping procedures in combination with specific glycosylhydrolases can be exploited (Chapter 51).
NMR Spectroscopy
NMR spectroscopy is a powerful tool capable of full de novo structural characterization of isolated glycans and simple glycan mixtures. Among its several advantages are its broad applicability (glycans contain an abundance of hydrogen (1H), the most easily detected magnetic nucleus), its nondestructive nature, the quantitative relationship between resonance intensity and residue concentration, the diverse set of experiments available, and the ability to return information on primary, secondary, and tertiary structure. Its principal limitation is sensitivity, typically requiring several nanomoles of glycan for even the simplest structure determination. Other limitations for both structural and metabolic applications are the relatively high cost of spectrometers and the level of expertise required for acquiring and interpreting NMR spectra. Nevertheless, there are emerging experiments using methods, such as dynamic nuclear polarization and looped, projected spectroscopy (L-PROSY), that provide several orders of magnitude improvement in sensitivity for applications that can monitor rapid in vivo conversions of metabolites such as glucose and fructose. Diffusion-based methods can also yield spectra of mixtures of components, separated by diffusion constants (effective molecular size).
When small amounts of sample are available and the source of the sample is known, the acquisition of a 1D 1H NMR spectrum, combined with simple 2D total correlation spectroscopy (TOCSY), can frequently identify a glycan. A 1D 1H NMR spectrum can be collected on ∼30 µg of a decasaccharide in ∼20 min on a state-of-the-art spectrometer (900 MHz with a cryoprobe). The anomeric 1H resonances are usually well-resolved in a relatively noncrowded region of the spectrum and show characteristic scalar couplings that are small for most α anomers and significantly larger for most β anomers. Thus, a first glance at the 1H -NMR spectrum gives a good estimate of how many residues there are (one anomeric 1H per residue except for Sia and KDOs) in a given oligosaccharide and how many of them belong to each anomeric type. NMR also provides a good estimate of how many residues are in a repeating unit of a polysaccharide. Connections from the anomeric (H1) resonances to the H2 and subsequently other resonances of a scalar coupled spin system can usually be seen in 2D TOCSY data, which show chemical shifts (resonance positions) for coupled 1Hs as crosspeaks on columns or rows emanating from an anomeric resonance (a ∼12-h acquisition for a 30-µg sample). The chemical shifts of two or more connected 1Hs are often sufficient to identify a residue. For example, the downfield (higher ppm) position and small couplings of three H2 resonances connected by crosspeaks to three α-anomeric resonances can identify these as belonging to core Man residues of an N-glycan. Historically, a collection of easily resolved resonances, often from 1D spectra, sensitive to both monosaccharide type and linkage type, were called reporter group resonances. These often prove sufficient to identify a glycan, particularly when options are restricted by knowledge of biosynthetic pathways in the system under study.
Today, it is possible to greatly extend glycan identification by including 13C chemical shifts correlated with directly bonded 1Hs using 2D heteronuclear single quantum coherence (HSQC) spectra (Figure. 50.3). 13C is magnetically active, but 13C spectroscopy is less sensitive than 1H spectroscopy. To improve sensitivity, 13C chemical shifts are indirectly detected through 1Hs. Even with the improved detection, 13C is only 1% naturally abundant, and unless the sample is isotopically enriched, approximately two orders of magnitude more material is required for collection of these data. Improvements in NMR hardware can reduce the quantities or time required and allow hybrid experiments, such as 2D HSQC-TOCSY, also illustrated in Figure 50.3. Furthermore, significant spectral overlap can be overcome with recent 2D NMR experiments optimized by using direct detection on 13C combined with optimized data processing. Web-based computational tools that rely on empirical rules or databases that correlate chemical shifts with structural features now exist, and these allow facile prediction of possible structures from 1D and 2D NMR data (e.g., CASPER).

FIGURE 50.3.
Sections of 2D 1H-13C 700 MHz NMR spectra of a sialyl Lewis x–capped glycan in D2O. In this example, the combination of heteronuclear single quantum coherence (HSQC) and HSQC-total correlation spectroscopy (TOCSY) spectra is used to assign the (more...)
When unprecedented structures emerge, it is also possible to perform a de novo structure determination using NMR data alone. This usually begins with a full assignment of the 1H and 13C resonances for each residue of a glycan using a combination of COSY, TOCSY, HSQC, and HSQC-TOCSY spectra. In addition, 2D heteronuclear multiple-bond correlation (HMBC or LR-HSQMBC) experiments are used to detect through-bond coupling between the anomeric 1H and the carbon atom on the opposite side of the glycosidic linkage. These are, however, even less sensitive and more time-consuming experiments. It is possible to avoid both 13C-based HSQC and HMBC experiments by substituting a 2D nuclear Overhauser effect (NOE) spectrum. In medium size glycans (1 kDa), NOE crosspeaks may go unobserved because of competition of coherence transfer pathways. Such crosspeaks can be observed using a rotating-frame Overhauser effect (ROE) spectrum. Both experiments correlate 1H resonances based on inter-1H distances, as opposed to through-bond couplings; they typically show crosspeaks for pairs of 1Hs that are within ∼4 Å of one another. Most connections are between 1Hs in the same residue, but typically an extra connection will be between an anomeric 1H and a 1H in another residue. This 1H is frequently across the glycosidic bond at the linkage site, providing a way to assemble residues into a complete structure. However, caution must be exercised. The identification of linkage sites is not as definitive as with 13C-based experiments, because the distance for the trans-glycosidic pair is dependent on glycosidic torsion angles, and these NOE crosspeaks can be weak. Also, other nonlinkage site protons can come within NOE distance and give false identification.
THREE-DIMENSIONAL GLYCAN STRUCTURE
The same NOE crosspeaks that assist in identifying linkages between residues can, in principle, be used to define the tertiary (3D) structure of a glycan. However, the interpretation is complicated by the presence of internal molecular motion, resulting in many different conformations in solution. This motion is nicely illustrated in ϕ–ψ energy plots much like the Ramachandran plots used to describe the energetics of torsional angles in the polypeptide backbone of a protein. For glycans, these plots are usually based on parameterized functions that represent a combination of quantum mechanical and experimental data. Such a plot is shown for a Glcβ1-4Glc linkage in Figure 50.4. The minima in these plots correlate well with angles found in structures of glycans deposited in databases such as the Cambridge Structural Database or the Protein Data Bank (PDB). Multiple minima indicate that multiple conformations are likely to be present in solution and therefore imply that there is conformational motion for the disaccharide. Thermal energies available to molecules at these temperatures are ∼0.6 kcal, so in solution, states within ∼1.4 kcal of the minimum have appreciable populations (>10%), representing a ±20° variation in torsion angles about those of the most stable form of the Glcβ1-4Glc linkage. In NMR experiments, intensities of NOE crosspeaks are roughly proportional to the average of the inverse sixth power of the distance sampled over these states, and the steep distance dependence results in skewing distances toward smaller numbers. Nevertheless, NOEs, particularly those representing close approach of remote parts of larger glycans, can guide construction of 3D structural models. Convenient tools for building structures are available, such as the carbohydrate builder of the GLYCAM package. Initial structures can be further refined with molecular mechanics or molecular dynamics software packages, or they can be manipulated in common molecular graphics packages to match solution data coming from NOEs and other NMR measurements, including residual dipolar couplings and paramagnetic effects, which provide angular and long range distance constraints respectively (Chapter 30).
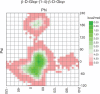
FIGURE 50.4.
Energy plots showing likely ϕ–ψ values for the glycosidic torsion angles between Glc residues in Glcβ1-4Glc-OMe. Regions in green indicate a likely combination of ϕ and ψ, or conformation, whereas red regions (more...)
ACKNOWLEDGMENTS
The authors acknowledge contributions to previous versions of this chapter by Carolyn R. Bertozzi and appreciate helpful comments and suggestions from Markus Pauly and Martina Delbianco.
FURTHER READING
- Jiménez-Barbero J, Peters T. 2003. NMR spectroscopy of glycoconjugates. Wiley-VCH, Weinheim, Germany.
- Lamari FN, Kuhn R, Karamanos NK. 2003. Derivatization of carbohydrates for chromatographic, electrophoretic and mass spectrometric structural analysis. J Chromatogr B Analyt Technol Biomed Life Sci 793: 15–36. doi:10.1016/S1570-0232(03)00362-3 [PubMed: 12880852] [CrossRef]
- Haslam SM, North SJ, Dell A. 2006. Mass spectrometric analysis of N- and O-glycosylation of tissues and cells. Curr Opin Struct Biol 16: 584–591. doi:10.1016/j.sbi.2006.08.006 [PubMed: 16938453] [CrossRef]
- Wuhrer M. 2013. Glycomics using mass spectrometry. Glycoconj J 30: 11–22. doi:10.1007/s10719-012-9376-3 [PMC free article: PMC3547245] [PubMed: 22532006] [CrossRef]
- Zaia J. 2013. Glycosaminoglycan glycomics using mass spectrometry. Mol Cell Proteomics 12: 885–892. doi:10.1074/mcp.R112.026294 [PMC free article: PMC3617335] [PubMed: 23325770] [CrossRef]
- Battistel MD, Azurmendi HF, Yu B, Freedberg DI. 2014. NMR of glycans: shedding new light on old problems. Prog Nucl Magn Reson Spectrosc 79: 48–68. doi:10.1016/j.pnmrs.2014.01.001 [PubMed: 24815364] [CrossRef]
- Balagurunathan K, Nakato H, Desai UR. eds. 2015. Glycosaminoglycans: chemistry and biology. In Methods in molecular biology, Vol. 1229. Springer, New York. [PubMed: 25489643]
- Yan H, Yalagala RS, Yan F. 2015. Fluorescently labelled glycans and their applications. Glycoconj J 32: 559–574. doi:10.1007/s10719-015-9611-9 [PubMed: 26239924] [CrossRef]
- Woods RJ. 2018. Predicting the structures of glycans, glycoproteins and their complexes. Chem Rev 118: 8005–8024. doi:10.1021/acs.chemrev.8b00032 [PMC free article: PMC6659753] [PubMed: 30091597] [CrossRef]
- Mucha E, Stuckmann A, Marianski M, Struwe WB, Meijer G, Pagel K. 2019. In-depth structural analysis of glycans in the gas phase. Chem Sci 10: 1272–1284. doi:10.1039/C8SC05426F [PMC free article: PMC6357860] [PubMed: 30809341] [CrossRef]
- Gimeno A, Valverde P, Ardá A, Jiménez-Barbero J. 2020. Glycan structures and their interactions with proteins. A NMR view. Curr Opin Struct Biol 62: 22–30. doi:10.1016/j.sbi.2019.11.004 [PMC free article: PMC7322516] [PubMed: 31835069] [CrossRef]
- Review Structural Analysis of Glycans.[Essentials of Glycobiology. 2015]Review Structural Analysis of Glycans.Mulloy B, Dell A, Stanley P, H. Prestegard J. Essentials of Glycobiology. 2015
- Chromatographic Profiling of N-Glycans.[Methods Mol Biol. 2019]Chromatographic Profiling of N-Glycans.Gohlke M, Blanchard V. Methods Mol Biol. 2019; 1934:65-81.
- Separation of N-glycans by HPLC.[Methods Mol Biol. 2008]Separation of N-glycans by HPLC.Gohlke M, Blanchard V. Methods Mol Biol. 2008; 446:239-54.
- Glycan analysis: scope and limitations of different techniques--a case for integrated use of LC-MS(/MS) and NMR techniques.[Anal Bioanal Chem. 2013]Glycan analysis: scope and limitations of different techniques--a case for integrated use of LC-MS(/MS) and NMR techniques.Fellenberg M, Behnken HN, Nagel T, Wiegandt A, Baerenfaenger M, Meyer B. Anal Bioanal Chem. 2013 Sep; 405(23):7291-305. Epub 2013 Jul 14.
- Review Structural Analysis of Glycans.[Essentials of Glycobiology. 2009]Review Structural Analysis of Glycans.Mulloy B, Hart GW, Stanley P. Essentials of Glycobiology. 2009
- Structural Analysis of Glycans - Essentials of GlycobiologyStructural Analysis of Glycans - Essentials of Glycobiology
Your browsing activity is empty.
Activity recording is turned off.
See more...