NCBI Bookshelf. A service of the National Library of Medicine, National Institutes of Health.
Varki A, Cummings RD, Esko JD, et al., editors. Essentials of Glycobiology [Internet]. 3rd edition. Cold Spring Harbor (NY): Cold Spring Harbor Laboratory Press; 2015-2017. doi: 10.1101/glycobiology.3e.019
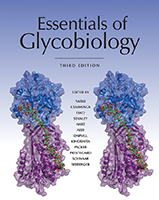
Essentials of Glycobiology [Internet]. 3rd edition.
Show detailsThis chapter presents an overview of the dynamic modification of serine or threonine hydroxyl moieties on nuclear, mitochondrial, and cytoplasmic proteins by O-linked β-linked N-acetylglucosamine, termed O-β-GlcNAc or simply O-GlcNAc. This seemingly simple carbohydrate modification plays key roles in cellular physiology and disease progression. Underpinning these observations are the thousands of O-GlcNAc-modified proteins that regulate cellular pathways such as epigenetics, gene expression, translation, protein degradation, signal transduction, mitochondrial bioenergetics, the cell cycle, and protein localization.
HISTORICAL BACKGROUND
O-GlcNAc was unexpectedly discovered in 1983, when purified bovine milk galactosyltransferase and its radiolabeled donor substrate (UDP-[3H]galactose) were used to probe for GlcNAc-terminating glycoconjugates on the surface of living murine thymocytes, splenic B- and T-lymphocytes, and macrophages. Galactosyltransferase is a Golgi glycosyltransferase that attaches galactose in a β1-4 linkage to almost any terminal N-acetylglucosamine residue (Chapter 6). Contrary to expectations, product analyses, including release from protein by alkali-induced β-elimination and resistance to cleavage by peptide-N-glycosidase F (PNGase F; Chapter 50), showed that most of the labeled galactosylated glycans existed within a minor fraction of broken cells, as single O-linked GlcNAc moieties (Figure 19.1). Studies of the subcellular localization of O-GlcNAc in rat liver established that O-GlcNAc is abundant within chromatin, concentrated on the nuclear pores of the nuclear envelope, and also present within the cytoplasm of the cell (Figure 19.2; Table 19.1).

FIGURE 19.1.
Many nuclear, mitochondrial, and cytoplasmic proteins are modified by monosaccharides of O-linked β-N-acetylglucosamine (O-GlcNAc). O-GlcNAc is dynamically added and removed from proteins by just two enzymes, the O-GlcNAc transferase (OGT) and (more...)

FIGURE 19.2.
(A) O-GlcNAcylated proteins occur in many different cellular compartments. Found mostly within the nucleus, they are also in the cytoplasm and mitochondria. The subcellular distribution of O-GlcNAc is similar to that of Ser(Thr)-O-phosphorylation. (Redrawn, (more...)
TABLE 19.1.
Some O-GlcNAcylated proteins
O-GlcNAc is distinct from other common forms of protein glycosylation in several respects: (1) it occurs mostly within the nuclear, mitochondrial, and cytoplasmic compartments of the cell; (2) the GlcNAc moiety is generally not elongated or modified to form more complex structures; and (3) O-GlcNAc is attached and removed multiple times in the life of a protein, often cycling rapidly on a protein. Of note, this modification is distinct from the recently described extracellular O-GlcNAc found on proteins containing epidermal growth factor (EGF) repeats (Chapter 13). The enzyme that catalyzes the addition of O-GlcNAc to EGF repeats (EOGT) is located in the endoplasmic reticulum (ER) and is closely related to the enzymes that catalyze mucin-like glycosylation. Unlike intracellular O-GlcNAcylation, modification of EGF repeats by O-GlcNAc is static and can be extended by the addition of galactose.
Why Did O-GlcNAc Remain Undetected for So Long?
Recent studies show that O-GlcNAc is found on thousands of nuclear, mitochondrial, and cytoplasmic proteins, including many heavily studied proteins such as RNA polymerase II, histones, and ribosomal proteins (Figure 19.2; Table 19.1). Yet, although phosphorylation was discovered in 1954, O-GlcNAc was not discovered until 1983. So why did O-GlcNAc remain undetected for so long? First, the long-standing standard dogma was that glycosylation (other than glycogen storage) did not occur with the nucleocytosolic compartment. Second, unlike charged modifications (e.g., phosphate), addition and removal of O-GlcNAc generally does not affect the migration of polypeptides on SDS-PAGE (sodium dodecyl sulfate polyacrylamide gel electrophoresis). A small shift in protein migration might be seen if the O-GlcNAc residues are highly clustered or if the protein is extensively O-GlcNAcylated at multiple sites (e.g., p62 nuclear pore protein). Third, all cells contain high levels of hydrolases, including abundant lysosomal hexosaminidases and nucleocytoplasmic β-N-acetylglucosaminidases, that rapidly remove O-GlcNAc from intracellular proteins when the cell is damaged or lysed. Thus, O-GlcNAc is often lost during the isolation of a protein. Finally, O-GlcNAc is particularly difficult to detect by physical techniques, such as mass spectrometry, because it often occurs at substoichiometric amounts on a protein and readily falls off the polypeptide during the ionization process in a mass spectrometer. In both electrospray ionization (ESI) mass spectrometry and in matrix-assisted laser desorption time of flight (MALDI-TOF) mass spectrometry analyses of a mixture of unmodified and O-GlcNAc–modified peptides, not only is the O-GlcNAc lost during ionization but the signal from the O-GlcNAc-modified peptides that remain is suppressed by the presence of the unmodified peptide. Our ability to detect O-GlcNAc has been improved in recent years by the development of monoclonal antibodies that detect O-GlcNAc, the use of “click chemistry” compatible sugars (Chapters 51 and 56), and the invention of more sophisticated mass spectrometric techniques, such as electron transfer dissociation (ETD) mass spectrometry.
ENZYMES CONTROLLING O-GlcNAc CYCLING
The dynamic cycling of O-GlcNAc on proteins is regulated by the concerted actions of enzymes encoded by just two genes: the O-GlcNAc transferase (OGT) and a neutral β-hexosaminidase known as O-GlcNAcase (OGA) (Figure 19.1). The regulation of the transcription start site and alternative splicing results in the synthesis of at least three isoforms of OGT and two isoforms of OGA. Nonetheless, the question remains: “how can so few enzymes specifically glycosylate and deglycosylate so many substrates?” Although both OGT and OGA each have only a single catalytic subunit, they actually exist within the cell as a multitude of different holoenzymes in which their catalytic subunits are noncovalently bound to a myriad of accessory proteins that appear to control their targeting (Figure 19.3).

FIGURE 19.3.
O-GlcNAc transferase (OGT) is regulated by multiple complex mechanisms, including transcriptional regulation of its expression, differential mRNA splicing, proteolytic processing, posttranslational modification, and multimerization with itself and other (more...)
O-GlcNAc Transferase
O-GlcNAc transferase (OGT; uridine diphospho-N-acetylglucosamine:polypeptide β-N-acetylglucosaminyltransferase; EC 2.4.1.255) catalyzes the addition of N-acetylglucosamine from UDP-GlcNAc to specific serine or threonine residues to form a β-O-glycosidic linkage. The enzyme was first identified and purified from rat liver and subsequently cloned from rat, human, Caenorhabditis elegans, and other organisms. OGT resides on the X chromosome (Xq13 in humans) near the centromere and is among the most highly conserved proteins from worms to man. To date, three isoforms of OGT have been well characterized: (1) the nucleocytoplasmic or full-length variant (ncOGT), which is 110 kDa; (2) a short isoform of OGT (sOGT), which is 78 kDa; and (3) a variant of OGT that is targeted to the mitochondria (mOGT; ∼90 kDa). In the nucleus and cytoplasm, OGT appears to form multimers, consisting of one or more 110-kDa subunits and 78-kDa subunits.
ncOGT has two distinct domains separated by a putative nuclear localization sequence. The amino terminus of each OGT subunit contains tetratricopeptide repeats (TPRs), which can number up to 12 (species dependent). The TPR domain serves as a protein–protein interaction domain and mediates interactions of the enzyme with substrates and targeting proteins (Figure 19.3). The major variation between the three (above) variants is in the number and sequence of the TPR repeats. The crystal structure of the human TPR domain of ncOGT shows that the repeats occur as stacked α-helical domains, forming a “tube-like” structure with a remarkable structural similarity to the armadillo repeat domain of the nuclear transport protein importin-α. The TPRs of OGT are also required for the multimerization of OGT subunits. The carboxy-terminal domain of OGT appears to have evolved from the glycogen phosphorylase superfamily of enzymes, and it contains the UDP-GlcNAc–binding and catalytic sites of the enzyme.
The regulation of OGT is quite complex and still not well understood. OGT is itself O-GlcNAcylated and also tyrosine-phosphorylated (Figure 19.3). Tyrosine phosphorylation appears to activate the enzyme, but the role of O-GlcNAc on OGT is not yet clear. Recent studies suggest that OGT is also targeted and regulated by Ser/Thr phosphorylation; AMPK, CAMKIV, and GSK3β have been reported to modify OGT altering either the localization or activity of the enzyme. Purified or recombinant OGT modifies small synthetic peptides based on known sites from O-GlcNAcylated proteins, but OGT appears to require accessory proteins to modify full-length protein substrates efficiently.
The high-energy nucleotide sugar used by OGT is UDP-GlcNAc, which is synthesized by the hexosamine biosynthetic pathway (HBP). Increased flux through the HBP, a result of increased glucose levels, has been shown to modulate O-GlcNAc levels (Figure 19.4). On transfer of GlcNAc to proteins, UDP is released, which is a potent feedback inhibitor of OGT. Under conditions during which UDP is rapidly removed (as occurs within the cell), OGT activity is dependent on the UDP-GlcNAc level over a remarkable range of concentrations (from the low nm range to well more than 50 mm). Of note, the substrate specificity of OGT appears to change at different UDP-GlcNAc concentrations, suggesting that OGT regulates cellular processes in a manner dependent on nutritional status.

FIGURE 19.4.
Elevating O-GlcNAc blocks insulin signaling at many points. Glucose flux via glucose transporters (e.g., GLUT4 in insulin-sensitive cells) through the hexosamine biosynthetic pathway (HBP; which accounts for 2%–5% of total glucose usage) leads (more...)
O-GlcNAcase
Nucleocytoplasmic β-N-acetylglucosaminidase (O-GlcNAcase; OGA; EC 3.2.1.169) was first identified as a neutral cytosolic hexosaminidase (referred to as “hexosaminidase C”). OGA was purified from rat kidney and bovine brain, and the human gene was cloned using peptide sequence information. The OGA gene was found to be identical to MGEA5, a putative hyaluronidase genetically identified because of its association with meningiomas. There are two well-characterized isoforms of OGA (short and full-length), which appear to arise from alternative splicing. Both variants contain an amino-terminal glycosidase domain related to hyaluronidases. The short OGA is identical to full-length OGA (916 amino acids) for the first 662 amino acids but possesses an alternative carboxy-terminal sequence 15 amino acids in length. Sequence analyses suggest that full-length OGA is a bifunctional enzyme, with the carboxyl terminus displaying homology with the GCN5 histone acetyl transferase (HAT) family. During the latter stages of apoptosis (regulated cell death), the executioner caspase (caspase-3) cleaves OGA to separate the HAT and OGA domains. Like OGT, OGA also interacts in a dynamic fashion with a very large number of cellular proteins, but the locations of interaction sites on the enzyme have not been determined.
O-GlcNAc IS A HIGHLY DYNAMIC MODIFICATION
Unlike the relatively static nature of mature N- and O-glycans on glycoproteins, O-GlcNAc cycles rapidly on and off most proteins. Early studies showed that mitogen or antigen activation of lymphocytes rapidly decreased O-GlcNAcylation of many cytoplasmic proteins, but concomitantly increased O-GlcNAcylation of many nuclear proteins. Likewise, neutrophils were shown to rapidly modulate O-GlcNAcylation of several proteins in response to chemotactic agents. Recently, changes in O-GlcNAc cycling have been shown in response to cellular stress, the cell cycle, neuron depolarization, and insulin signaling. Pulse-chase analyses have revealed that O-GlcNAc residues on small heat-shock protein in the lens (α-crystallin) and on intermediate filament proteins (cytokeratins) turn over more rapidly than the polypeptide chains to which they are attached. These observations, combined with those demonstrating that OGT uses amino acid motifs similar to proline-directed mitogen-activated protein (MAP) kinases, suggest that O-GlcNAc is a regulatory posttranslational modification analogous to phosphorylation.
O-GlcNAc IS UBIQUITOUS AND ESSENTIAL IN METAZOANS
To date, nucleocytoplasmic O-β-GlcNAc has been found in all multicellular organisms investigated, ranging from filamentous fungi, worms, insects, and plants to humans. Recent data suggests that the O-GlcNAc modification is also found in a subset of prokaryotes such as Listeria monocytogenes. It is currently unclear if the enzymes responsible for this modification are related to OGT or EOGT. To date, O-GlcNAc and the enzymes that control its cycling do not appear to exist in yeast, such as Saccharomyces cerevisiae or Schizosaccharomyces pombe. However, recently, it was discovered that S. cerevisiae have O-mannose moieties on the same proteins and at the same sites where O-GlcNAc is found in multicellular organisms. O-α-GlcNAc appears to be a common modification of cell-surface and extracellular proteins in protozoa, where it is attached by enzymes structurally related to the lumenal protein:O-GalNAc transferases (ppGalNAc-Ts) in mammals (Chapter 10). The presence of the O-α-GlcNAc modification has complicated the analysis of O-β-GlcNAc in protozoa; however, O-GlcNAc and OGT have been reported in Giardia lamblia and Cryptosporidium parvum. O-GlcNAc–modified proteins have also been found on many viruses that infect metazoans (e.g., adenovirus, SV40, cytomegalovirus, rotavirus, baculovirus, plum pox, HIV, and others). In viruses, instead of being localized on the outside capsid of the virus where “classical” N- or O-glycans are found (Chapter 42), O-GlcNAc is found deep within the viruses, on tegument and other regulatory proteins, close to the nucleic acid components.
Genetic and subsequent biochemical studies in Arabidopsis thaliana showed that the genes SPY and SECRET AGENT regulate growth hormone (gibberellic acid) signaling, and later studies established that both of these genes encode O-β-GlcNAc transferases. Mutations in either SPY or SECRET AGENT cause severe growth phenotypes, but they are not lethal. However, simultaneous mutation of both genes is lethal. Studies in rice, potatoes, and other plants have also indicated that O-GlcNAcylation is important for growth regulation. Unlike plants, mammals and insects appear to have only a single gene encoding the catalytic subunit of the OGT. Using Cre-LoxP conditional gene disruption in mice, OGT was shown to be required for the viability of embryonic stem cells. Tissue-targeted disruption in mice and disruption of OGT expression in cell culture have established that O-GlcNAcylation is essential for viability at the single-cell level in mammalian cells. Disruption of OGT in the worm C. elegans causes defective carbohydrate metabolism, abnormalities in dauer formation, and reduced life span. In Drosophila, homozygous mutants survive for >5 days until the late larval stage, but subsequently die in the pupal cases. Targeted, inducible deletion of OGT in αCAMKII-positive (excitory) neurons in brains of adult mice rapidly results in morbidly obese mice because of defects in satiety, further supporting the roles of O-GlcNAcylation as a nutrient sensor. The highly homologous and conserved nature of OGT among metazoans allows a transgene encoding the human sequence of OGT to be used to rescue OGT null Drosophila melanogaster. Recently, OGA has been disrupted in both C. elegans and mammalian models. Like the OGT null, the C. elegans OGA null has defective carbohydrate metabolism and abnormalities in dauer formation. In contrast to the OGT null, in C. elegans, in which OGA has been disrupted, the worms have an extended life span. In murine models, deletion of OGA leads to perinatal lethality that is associated with genomic instability and severe metabolic phenotypes. The OGA heterozygote mouse shows widespread changes in transcription and metabolism, and an enhanced dependence on glucose metabolism.
O-GlcNAc Is Found on a Wide Range of Proteins
Studies from several laboratories have described O-GlcNAcylated proteins from virtually all cellular compartments representing nearly all functional classes of protein (Figure 19.2; Table 19.1). O-GlcNAc is particularly abundant within the nucleus, where it occurs on the transcriptional regulatory machinery including RNA polymerase II catalytic subunit carboxy-terminal domain (CTD) and basal, as well as other, transcription factors, histones, and DNA methyltransferases. O-GlcNAcylation also appears to be particularly abundant on proteins involved in signaling, stress responses, and energy metabolism. Nearly 90 proteins within the mitochondria are dynamically O-GlcNAcylated with the highest concentration found within the electron transport chain. It also occurs on many cytoskeletal regulatory proteins, such as those regulating actin assembly (e.g., vinculin, talin, vimentin, and ankyrin) and tubulin assembly (e.g., MAPs, dynein, and tau). Even α-tubulin itself is dynamically modified by O-GlcNAc, but the stoichiometry appears to be low. Intermediate filaments, such as cytokeratins and neurofilaments in brain, are also heavily modified by O-GlcNAc.
Individual sites modified by O-GlcNAc have been identified on numerous proteins. Although there is no consensus motif that dictates glycosylation by OGT, proline and valine residues are common on either side of the modified hydroxyl amino acid. The other O-GlcNAc sites seemingly have little in common at the primary sequence level, but they have sequences similar to those also recognized by different kinases. Many of the identified O-GlcNAc sites have high “PEST” scores, a sequence motif that is associated with rapid degradation of a protein. A few studies suggest that O-GlcNAcylation within the PEST sequence prevents rapid degradation of the protein.
O-GlcNAc HAS A COMPLEX DYNAMIC INTERPLAY WITH O-PHOSPHATE
Site-mapping studies have shown that protein kinases and OGT can use the same serine and threonine residues, suggesting that a complex interplay exists between these two posttranslational modifications that fine tunes signal transduction networks. In fact, major enzymes that remove O-phosphate, protein phosphatase 1 β and γ, are in a dynamic complex with OGT indicating that in many cases the same enzyme complex both removes O-phosphate and concomitantly attaches O-GlcNAc (Figure 19.1). One example of a protein in which phosphorylation and O-GlcNAc appear competitive is the CTD repeat domain (YSPTSPS) of RNA polymerase II, which can contain as many as three O-GlcNAc residues per repeat. After the initiation step of the transcription cycle, O-GlcNAc on the CTD is removed and replaced with O-phosphate, initiating the elongation phase of transcription. In vitro studies have shown that synthetic peptides with up to ten CTD repeats (comprising 70 amino acids) cannot be phosphorylated by CTD kinases if they contain even a single O-GlcNAc moiety. Likewise, these CTD peptides cannot be O-GlcNAcylated if even one of the repeats contains a single O-phosphate residue.
On some proteins (e.g., casein kinase II), O-GlcNAc and O-phosphate occur at separate but adjacent sites, yet they still appear to be reciprocal with each other. However, on other proteins, the relationship between O-GlcNAc and O-phosphate dynamics remains unclear. For example, on cytokeratins, O-GlcNAcylation and O-phosphorylation appear to be independently regulated, but may occur mutually exclusively on completely different subsets of the same polypeptides. Complicating this relationship further, recent data has shown that many kinases are O-GlcNAc-modified and that glycosylation can alter the activity and their association with substrates.
BIOLOGICAL FUNCTIONS OF O-GlcNAc
Like O-phosphorylation, the specific functions of O-GlcNAc depend on the protein and sites to which the moiety is attached. However, some generalities are emerging. As discussed above, one of the major functions of O-GlcNAc is to prevent O-phosphorylation and, by doing so, to modulate signaling and transcription in response to cellular nutrients or stress.
O-GlcNAc Regulates Epigenetics and Transcription
Genome-wide studies have shown that OGT, OGA, and O-GlcNAc are found on thousands of promoters in C. elegans. Deletion of either OGT or OGA has a profound, but complicated effect, on transcription. Consistent with key roles for O-GlcNAc, deletion of OGT from C. elegans (L1 stage) results in the up-regulation of 299 transcripts and the suppression of 389 transcripts; whereas deletion of OGA results in the up-regulation of 218 transcripts and the suppression 291 transcripts. These complicated effects likely result from the observation that RNA polymerase II and the basal transcription complex are O-GlcNAc-modified. Moreover, O-GlcNAc directly regulates the activities of a variety of transcription factors including Sp1, estrogen receptors, STAT5 (signal transducer and activator of transcription 5), NF-κB (nuclear factor- κB), p53, YY1 (Yin Yang 1), Elf-1 (E74-like factor 1), c-Myc, Rb (retinoblastoma), PDX-1 (pancreatic and duodenal homeobox 1), CREB (cAMP response element binding), forkhead, and others.
In addition to modulating transcription directly, O-GlcNAc has been strongly implicated in mediating epigenetics. For instance, recent studies have shown that histones (H2A, H2B, H3, and H4) are modified by O-GlcNAc. Moreover, many epigenetic regulators are themselves O-GlcNAc-modified or associate with OGT/OGA. OGT associates with the ten-eleven translocation (TET) proteins, mSin3A/HDAC complexes, and the Polycomb group (PcG) proteins that regulate DNA methylation. In Drosophila, OGT, which is allelic with super sex combs (sxc), plays a role in Polycomb repression. The association of OGT with the PRC2 complex is critical in maintaining methylation of DNA and suppressing transcription.
O-GlcNAc Regulates Protein Translation, Stability, and Turnover
O-GlcNAc appears to modulate various stages of protein expression, stability, and turnover. First, at least 15 well-characterized ribosomal proteins and several associated translational factors are O-GlcNAcylated. It has been proposed that the activity of eukaryotic initiation factor 2 (eIF2) is regulated by its binding to p67. The p67 protein is O-GlcNAcylated, and the interaction between O-GlcNAcylated p67 and eIF2 prevents phosphorylation, thus promoting translation. Second, O-GlcNAc has been reported to prevent protein aggregation, and this is important in models of neurodegenerative disease and during injury. Third, O-GlcNAcylation of proteins has been reported to increase their half-life. Reports suggest that this is due to increased stability of recently translated proteins, suppression of proteasome activity, and reduced targeting of proteins for degradation. Last, it appears that the 26S proteasome itself is O-GlcNAc-modified. Recent proteomic analyses of the 26S proteasome have shown that 5 of 19 and 9 of 14 proteins of the catalytic and regulatory cores, respectively, are modified by O-GlcNAc. Increased O-GlcNAcylation of the Rpt2 ATPase, a component of the 19S cap of the proteasome, blocks its ATPase activity, reducing proteasome-catalyzed degradation. It has been suggested that O-GlcNAcylation of the proteasome allows the cell to respond to metabolic needs by controlling the availability of amino acids and altering the half-lives of key regulatory proteins.
O-GlcNAc Is Involved in Neurodegenerative Disease
Impaired glucose metabolism has been linked to the onset of several neurodegenerative diseases, and one feature is the reduced O-GlcNAcylation of key proteins. OGT and OGA map to loci linked to Parkinson's dystonia and late-onset Alzheimer's disease (AD), suggesting that changes in the expression and activity of these enzymes may contribute to the onset of neurodegenerative diseases. Consistent with a model in which decreased O-GlcNAcylation exacerbates the side effects of AD, as well as frontotemporal dementia and parkinsonism, increasing O-GlcNAc levels artificially reduces plaque formation and improves cognition in murine models.
AD is characterized by the production and oligomerization of amyloid peptide β1-42, which is derived from proteolytic processing of amyloid-β precursor protein. The appearance of amyloid peptide in cerebrospinal fluid is concomitant with a reduction in glucose metabolism and hyperphosphorylation and oligomerization of tau. Hyperphosphorylated tau is ultimately secreted into the cerebrospinal fluid, where it aggregates to form toxic neurofibrillary tangles (PHF [paired helical filament]-tau). These events precede neurodegeneration and brain atrophy. At a molecular level, O-GlcNAc is thought to counteract the effects of AD at several points. First, O-GlcNAcylation of γ-secretase suppresses its activity, reducing the production of amyloid peptide β1-42. Second, on tau, O-GlcNAcylation and phosphorylation appear reciprocal. Recent studies on tau have established that O-GlcNAcylation negatively regulates its O-phosphorylation in a site-specific manner both in vitro and in vivo. These data suggest that O-GlcNAcylation can suppress phosphorylation of tau, and thus reduce the formation of the toxic PHF-tau. Last, O-GlcNAcylated tau appears less likely to aggregate in vitro when compared with unmodified tau. These data suggest that not only does O-GlcNAc prevent toxic hyperphosphorylation of tau, but that O-GlcNAc stabilizes tau protein structure. Drugs that inhibit O-GlcNAcase to raise O-GlcNAc are in clinical trials for AD.
Altered O-GlcNAcylation has been reported for other proteins involved in neurodegenerative disease. Neurofilaments appear to be hypo-O-GlcNAcylated in neurons from patients with Lou Gehrig's disease (ALS [amyotrophic lateral sclerosis] and MND [motor neuron disease]). Clathrin-assembly proteins AP-3 and AP-180 are both modified by O-GlcNAc, and these modifications decline in AD, suggesting that reduced O-GlcNAc is associated with the loss of synaptic vesicle recycling. Altogether, the current data points to potentially significant roles of the O-GlcNAc modification in normal neuronal function and in the molecular mechanisms underlying the pathology of neurodegenerative disease.
Elevated O-GlcNAc Underlies Diabetes and Glucose Toxicity
Perhaps the best-understood function of O-GlcNAc is its role in the regulation of insulin signaling and as a mediator of glucose toxicity (see Figure 19.4). The HBP is in a unique position to sense nutrients, coordinating cellular metabolism in response to nucleotide levels, acetyl-CoA, nitrogen metabolism (glutamine), and glucose levels. Flux through the HBP and subsequent changes in O-GlcNAc levels are thought to mediate signaling pathways, inducing an appropriate response from cells given their nutritional state. For instance, increased O-GlcNAcylation of PDX-1, a pancreatic β-cell transcription factor that controls insulin transcription, increases its affinity for DNA and results in increased proinsulin transcription.
The first studies to link glucosamine metabolism directly with the toxicity of glucose in diabetes showed that glucosamine is many times more potent than glucose in inducing insulin resistance, one hallmark of type II diabetes, in cultured adipocytes. These studies also showed that the ability of glucose to induce insulin resistance could be blocked by deoxynorleucine (DON), a drug that inhibits glutamine:fructose amidotransferase (GFAT, the enzyme that converts fructose-6-P to glucosamine-6-P), and that this blockage could be bypassed by adding glucosamine to the culture media (Chapter 5). In 2002, two seminal studies suggested that aberrant O-GlcNAcylation, a result of elevated UDP-GlcNAc levels, is one molecular mechanism by which glucose and glucosamine metabolism led to insulin resistance. Collectively, these studies showed that artificially increasing O-GlcNAcylation in adipocytes or muscle blocks insulin signaling at several points, and overexpression of OGT in muscle or adipose tissue in transgenic mice causes insulin resistance and hyperleptinemia. Recent data shows that overexpression of OGT in the liver also induces insulin resistance and dyslipidemia. Consistent with these data, overexpression of OGA in the liver rescues circulating glucose levels in diabetic mice. Aberrant O-GlcNAcylation is also associated with many of the complications arising from Type 2 diabetes mellitus. For instance, inappropriate O-GlcNAcylation is associated with mitochondrial dysfunction and contractile defects in the hearts of diabetic mice. Based on these and other studies, elevated levels of O-GlcNAc have been characterized in several models of diabetes and are being investigated as a marker of prediabetes.
O-GlcNAcylation and Cancer
The Warburg effect describes a common metabolic phenotype of cancer cells in which anaerobic glycolysis is used in preference to oxidative phosphorylation. One consequence of this phenotype is increased glucose transport and subsequently increased flux of metabolites through the HBP. Recent studies suggest that one outcome of the Warburg effect is increased O-GlcNAcylation (prostate, breast, lung, colon, and liver) and that these changes in glycosylation modulate signaling pathways, metabolism, and transcriptional profiles. Collectively, changes in O-GlcNAcylation are thought to promote resistance to cell death stimuli and augmentation of angiogenesis, invasion, metastasis, and proliferation, thus enhancing the cancer cell phenotype. Supporting these data is the fact that suppressing the activity of OGT reduces proliferation and migration of cancer cells. Numerous mechanisms have been reported, and these changes in O-GlcNAcylation target changes in transcription, metabolism, and signaling. For example, O-GlcNAcylation of phosphofructokinase 1 (PFK1) shifts metabolites into the pentose phosphate pathway, augmenting glutathione levels and thus enhancing the ability of cancer cells to withstand oxidative stress. Together, these data suggest that detection of key O-GlcNAcylated proteins and expression of OGT/OGA may provide novel biomarkers for the early detection of human cancer. Given the dependence of cancer cells on O-GlcNAc, targeting OGT and other components of the HBP may reduce the aggressiveness of cancer cells while sensitizing them to chemotherapeutic drugs.
O-GlcNAc and Cellular Stress Survival
In every mammalian cell type examined to date, cellular stress initiates a signal that results in a rapid and global increase in O-GlcNAcylation on a multitude of proteins. Levels of O-GlcNAc increase rapidly in response to cellular injury in both in vitro (heat shock, ethanol, UV, hypoxia/reoxygenation, reductive, oxidative, and osmotic stress) and in vivo (ischemia preconditioning and remote ischemic preconditioning) models. Dynamic changes in the O-GlcNAc modification appear to result from changes in the activity and expression of OGT and OGA, as well as flux through the HBP. Several lines of evidence suggest that stress-induced elevations in O-GlcNAcylation promote a survival signaling program in cells and tissues: (1) suppressing O-GlcNAc levels, pharmacologically and genetically, sensitizes cells to oxidative, osmotic, and heat stress; and (2) elevating O-GlcNAc levels, pharmacologically and genetically, promotes survival in models of heat stress, hypoxia reoxygenation, oxidative stress, trauma hemorrhage, and ischemic reperfusion injury of the heart. Consistent with these observations, artificial modulation of O-GlcNAc levels appears to target pathways known to modulate cellular survival. For instance, in models of heat stress, dynamic O-GlcNAcylation promotes the induction of heat shock proteins, chaperones that promote survival by refolding proteins and inhibiting proapoptotic pathways. In models of myocardial infarction (heart attack), elevating O-GlcNAcylation has been shown to suppress all of the hallmarks of ischemia reperfusion injury, and include mitochondrial dysfunction, ER stress, increased reactive oxygen species, opening of the mitochondrial permeability transition pore, and calcium overload. Despite these compelling data, our understanding of the molecular events that underlie these observations remains unclear. Elucidating how increasing O-GlcNAcylation helps a cell to survive stressful conditions should provide novel targets for the development of therapeutics for treating conditions such as stroke and myocardial infarction.
FUTURE DIRECTIONS
During the past three decades, O-GlcNAcylation has been shown to be a sensor of cellular state (nutrition, stress, cell-cycle state) regulating or modulating nearly every cellular process, including signaling, transcription, translation, cytoskeletal functions, and cell division. O-GlcNAc plays critical roles in chronic diseases of aging, including diabetes, cancer, neurodegeneration, and cardiomyopathies. However, the global importance of O-GlcNAc remains unappreciated by most researchers. The modification is difficult to detect by standard biochemical methods, it is labile in mass spectrometers, and there is a profound lack of facile tools to study its biological functions. Improved methods for site-mapping O-GlcNAc, methods for altering its stoichiometry at single sites on proteins, and site-specific antibodies for hundreds of key proteins will be required if we are to understand the biological significance of this essential and ubiquitous protein modification. Elucidation of O-GlcNAc's roles in glucose toxicity in diabetes and in mechanisms of neoplasia and its functions in neurons are key future areas of investigation. After 30 years of investigation, we are still only beginning to understand the remarkable significance of O-GlcNAcylation in all aspects of eukaryotic biology.
ACKNOWLEDGMENTS
The authors appreciate helpful comments and suggestions from Jarrod W. Barnes, Albert Lee, and Krithika Vaidyanathan.
FURTHER READING
- Torres C-R, Hart GW. 1984. Topography and polypeptide distribution of terminal N-acetylglucosamine residues on the surfaces of intact lymphocytes. Evidence for O-linked GlcNAc. J Biol Chem 259: 3308–3317. [PubMed: 6421821]
- Hart GW. 1997. Dynamic O-linked glycosylation of nuclear and cytoskeletal proteins. Annu Rev Biochem 66: 315–335. [PubMed: 9242909]
- Comer FI, Hart GW. 2000. O-Glycosylation of nuclear and cytosolic proteins. Dynamic interplay between O-GlcNAc and O-phosphate. J Biol Chem 275: 29179–29182. [PubMed: 10924527]
- Wells L, Vosseller K, Hart GW. 2001. Glycosylation of nucleocytoplasmic proteins: Signal transduction and O-GlcNAc. Science 291: 2376–2378. [PubMed: 11269319]
- Vocadlo DJ, Hang HC, Kim EJ, Hanover JA, Bertozzi CR. 2003. A chemical approach for identifying O-GlcNAc-modified proteins in cells. Proc Natl Acad Sci 100: 9116–9121. [PMC free article: PMC171382] [PubMed: 12874386]
- Liu F, Iqbal K, Grundke-Iqbal I, Hart GW, Gong CX. 2004. O-GlcNAcylation regulates phosphorylation of tau: A mechanism involved in Alzheimer's disease. Proc Natl Acad Sci 101: 10804–10809. [PMC free article: PMC490015] [PubMed: 15249677]
- Love DC, Hanover JA. 2005. The hexosamine signaling pathway: Deciphering the “O-GlcNAc code”. Science STKE 312: re13. [PubMed: 16317114]
- Slawson C, Housley MP, Hart GW. 2006. O-GlcNAc cycling: How a single sugar posttranslational modification is changing the way we think about signaling networks. J Cell Biochem 97: 71–83. [PubMed: 16237703]
- Groves JA, Lee A, Yildirir G, Zachara NE. 2013. Dynamic O-GlcNAcylation and its roles in the cellular stress response and homeostasis. Cell Stress Chaperones 18: 535–558. [PMC free article: PMC3745259] [PubMed: 23620203]
- Dassanayaka S, Jones SP. 2014. O-GlcNAc and the cardiovascular system. Pharmaco Ther 142: 62–71. [PMC free article: PMC3943723] [PubMed: 24287310]
- Hardivillé S, Hart GW. 2014. Nutrient regulation of transcription, signaling, and cell physiology by O-GlcNAcylation. Cell Metab 20: 208–213. [PMC free article: PMC4159757] [PubMed: 25100062]
- Lewis BA, Hanover JA. 2014. O-GlcNAc and the epigenetic regulation of gene expression. J Biol Chem 289: 34440–34448. [PMC free article: PMC4263851] [PubMed: 25336654]
- Ma Z, Vosseller K. 2014. Cancer metabolism and elevated O-GlcNAc in oncogenic signaling. J Biol Chem 289: 34457–34465. [PMC free article: PMC4263853] [PubMed: 25336642]
- Vaidyanathan K, Wells L. 2014. Multiple tissue-specific roles for the O-GlcNAc posttranslational modification in the induction of and complications arising from type II diabetes. J Biol Chem 289: 34466–34471. [PMC free article: PMC4263854] [PubMed: 25336652]
- Zhu Y, Shan X, Yuzwa SA, Vocadlo DJ. 2014. The emerging link between O-GlcNAc and Alzheimer disease. J Biol Chem 289: 34472–34481. [PMC free article: PMC4263855] [PubMed: 25336656]
- Halim A, Larsen IS, Neubert P, Joshi HJ, Petersen BL, Vakhrushev SY, Strahl S, Clausen H. 2015. Discovery of a nucleocytoplasmic O-mannose glycoproteome in yeast. Proc Natl Acad Sci 112: 15648–15653. [PMC free article: PMC4697373] [PubMed: 26644575]
- Ma J, Liu T, Wei AC, Banerjee P, O'Rourke B, Hart GW. 2015. Protein O-GlcNAcylation regulates cardiac mitochondrial function. J Biol Chem 290: 29141–29153. [PMC free article: PMC4705920] [PubMed: 26446791]
- Lagerlöf O, Blackshaw S, Hart GW, Huganir RL. 2017. The nutrient sensor OGT regulates feeding in αCaMKII-positive neurons of the PVN. Science 351: 1293–1296. [PMC free article: PMC4817221] [PubMed: 26989246]
- Review The O-GlcNAc Modification.[Essentials of Glycobiology. 2022]Review The O-GlcNAc Modification.Zachara NE, Akimoto Y, Boyce M, Hart GW. Essentials of Glycobiology. 2022
- Review The O-GlcNAc Modification.[Essentials of Glycobiology. 2009]Review The O-GlcNAc Modification.Hart GW, Akimoto Y. Essentials of Glycobiology. 2009
- O-GlcNAcylation of Neuronal Proteins: Roles in Neuronal Functions and in Neurodegeneration.[Adv Neurobiol. 2014]O-GlcNAcylation of Neuronal Proteins: Roles in Neuronal Functions and in Neurodegeneration.Lagerlöf O, Hart GW. Adv Neurobiol. 2014; 9:343-66.
- Brain O-GlcNAcylation: From Molecular Mechanisms to Clinical Phenotype.[Adv Neurobiol. 2023]Brain O-GlcNAcylation: From Molecular Mechanisms to Clinical Phenotype.Uygar B, Lagerlöf O. Adv Neurobiol. 2023; 29:255-280.
- O-Linked N-Acetylglucosamine Transiently Elevates in HeLa Cells during Mitosis.[Molecules. 2018]O-Linked N-Acetylglucosamine Transiently Elevates in HeLa Cells during Mitosis.Fisi V, Kátai E, Orbán J, Dossena S, Miseta A, Nagy T. Molecules. 2018 May 26; 23(6). Epub 2018 May 26.
- The O-GlcNAc Modification - Essentials of GlycobiologyThe O-GlcNAc Modification - Essentials of Glycobiology
Your browsing activity is empty.
Activity recording is turned off.
See more...