NCBI Bookshelf. A service of the National Library of Medicine, National Institutes of Health.
Menini A, editor. The Neurobiology of Olfaction. Boca Raton (FL): CRC Press/Taylor & Francis; 2010.
8.1. INTRODUCTION
The initial steps of olfaction occur in primary sensory neurons located in the olfactory epithelium of the nasal cavity of vertebrates. These neurons are responsible for the detection of odorant molecules present in the surrounding environment and the generation of the neural signal that is transmitted to the brain. The morphology of the primary sensory neurons was described by Max Schultze in the second half of the nineteenth century (for review, see Zippel 1993), but only about 100 years later the first reviews describing some functional properties of these neurons were published (Getchell 1986; Lancet 1986). Primary sensory neurons of the olfactory epithelium, often indicated by various names: olfactory receptor cells (ORCs), olfactory sensory neurons (OSNs), or olfactory receptor neurons (ORNs), are bipolar neurons with a single dendrite that terminates with a knob, from which several tiny cilia protrude, where the transduction of the olfactory signal takes place. Odorant molecules bind to odorant receptors, and this interaction triggers an increase in the intraciliary concentration of cyclic adenosine monophosphate (cAMP) through the activation of the receptor-coupled G-protein and adenylyl cyclase (AC). Cyclic nucleotide-gated (CNG) channels located in the ciliary membrane are directly activated by cytoplasmic cAMP, causing a depolarizing influx of Na+ and Ca+ ions. The odorant-induced inward transduction current has been shown to be composed not only of a cation influx through CNG channels, but also of a C– efflux through C– channels activated by Ca2+ (Cl(Ca) channels). This chapter will review the molecular mechanisms underlying the functional role of vertebrate olfactory cilia.
8.2. ANATOMICAL GEOMETRY OF THE VERTEBRATE OLFACTORY RECEPTOR CELL (ORC) AND SENSORY CILIA
In the nose of vertebrates, odorants are detected by the main olfactory epithelium located in the nasal cavity (Figure 8.1A). The main olfactory epithelium is composed of several types of populations of sensory cells. The reader is referred to Chapter 9 of this book for a detailed discussion of subsystems of sensory cells (see also Tirindelli et al. 2009). The three main types of cells are the principal ORCs, the supporting cells, and the basal cells. ORCs are bipolar neurons with a single dendrite from which tens of cilia elongate into the olfactory mucus that covers the surface of the epithelium (Menco and Morrison 2003), and a single axon that projects directly to the olfactory bulb of the brain.
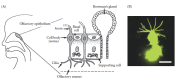
FIGURE 8.1
Olfactory epithelium and olfactory receptor cell. (A) Schematic diagram of the localization of the human olfactory epithelium in the upper part of the nasal cavity (left panel). Schematic diagram showing the histological organization of the olfactory (more...)
At the apical part of the dendrite, ORCs form tight junctions with the neighboring cells, mainly with supporting cells. Therefore, ORCs are in contact with different subregions defined by the surrounding environments. The apical region faces the outside of the body and is directly exposed to odorants, while the other parts of the neuron are embedded in the epithelium and are surrounded by the interstitial solution. Since the former is directly exposed to the external environment, the mucosal ionic condition (see Table 8.1) could be affected by the external conditions, such as the humidity. Possible changes in the ionic environment will be especially relevant for water-living animals. In contrast, the ionic composition of the interstitial solution is expected to be stable.
TABLE 8.1
Intracellular and Extracellular Ion Concentrations at the Apical Part of ORCs and Calculated Nernst Potentials.
At the apical part of the ORCs, the dendritic tip is slightly swelled into a “terminal swelling” or “olfactory knob,” from which cilia extend to expose their membrane to the external environment. The ORCs of lower vertebrates have about six motile cilia that can be as long as 200 u.m (Menco 1980). The diameter of a frog cilium is about 0.28 μm near the base of the cilium and becomes 0.19 μm in the distal portion (Menco 1980). The ORCs of mammals have about 17 nonmotile cilia, ranging from 15 to 50 αm in length, with a diameter of about 0.11 αm in their distal portion (Lidow and Menco 1984; Menco 1997, 1980; Menco and Morrison 2003). The ciliary structure allows two important features: on one side, the presence of numerous fine cilia protruding from the olfactory knob greatly increases the surface area of the ORC that can be exposed to the external environment (Menco 1980) and produces an increase in the probability of interaction with odorant molecules, and on the other side, the long and thin structure of each cilium increases the ratio between the membrane surface area and the cytoplasmic volume. This feature is of great relevance in the transduction process, because in a small volume a limited variation in the number of molecules could produce a large concentration change. It should also be taken into account that the volume of the cilia available for the cytosol is further restricted by the ciliary cytoskeleton. Indeed, the cilia contain the 9+2 microtubules structure and toward the tip, where the diameter of cilia becomes thinner, its structure changes by lacking the surrounding nine microtubules (Kerjaschki 1976).
8.3. FUNCTIONS OF THE OLFACTORY CILIA
Several important physiological functions occur in the olfactory cilia, including odorant detection, generation of electrical excitation, signal amplification, adaptation or desensitization, and masking. An outline of each of these functions will be described in this section, while the molecular mechanisms underlying each of them will be discussed in the following sections.
8.3.1. Electrical Excitation
The origin of odorant perception is the chemical interaction of odorant molecules with ORCs that convert the chemical information into electrical signals carrying information about the external world to the brain (for detailed reviews, see Schild and Restrepo 1998; Kleene 2008). In isolated ORCs, the response to odorant stimuli in solution has been well characterized. The response has mostly been measured under voltage-clamp upon presentation of a brief pulse of odorant. As illustrated in Figure 8.2A, the odorant stimulation generates a transient inward receptor current that is expected to depolarize the neuron in situ. The response typically lasts 1 s or more. In amphibians, the latency between the arrival of the stimulus and the onset of the current ranges from 150 to 600 ms (Firestein et al. 1990, 1993; Firestein and Werblin 1987; Kurahashi 1989; Tomaru and Kurahashi 2005; Takeuchi and Kurahashi 2003). In the mouse and rat, the latency is at most 160 ms (Reisert and Matthews 2001; Grosmaitre et al. 2006). This shorter latency is observed even in the intact epithelium, which requires that odorants diffuse through the mucus (Grosmaitre et al. 2006). For a strong stimulus, the amplitude of the peak receptor current can reach 1 nA (Ma et al. 2003; Firestein et al. 1993; Lowe and Gold 1993). The resting membrane potential of the ORC is between −80 and −60 mV (see discussion, Lagostena and Menini 2003) and the transduction current induces a slow and graded receptor potential. When the receptor potential reaches the threshold level, it induces action potentials. Figure 8.2B shows action potentials in response to an odorant stimulus in an ORC in the current-clamp configuration. The amplitude of the receptor potential becomes larger as the odorant concentration increases, producing a higher frequency of action potentials that send information about the odorant concentration to the olfactory bulb (Ma et al. 1999; Imanaka and Takeuchi 2001).

FIGURE 8.2
Voltage and current responses induced by odorant stimulation. (A) Current responses evoked by odorant stimulation under the voltage-clamp condition with holding potential at –60 mV. After a short latency, the odorant stimulation induced a transient (more...)
Olfactory cilia were first indicated as the site of odorant detection on the basis of their location at the interface between the internal and the external side of the body and of their morphology (Usukura and Yamada 1978; Menco 1980). Subsequent physiological studies have clearly shown that the cilia play a central role for odorant detection (Kurahashi 1989; Takeuchi and Kurahashi 2008; Firestein et al. 1990; Lowe and Gold 1991). As illustrated in Figure 8.3, an odorant stimulus directed at the cilia of an isolated ORC produced large responses, whereas stimuli directed at the soma gave very small responses (Kurahashi 1989). Moreover, it has been shown that the molecular elements needed for olfactory transduction are concentrated at the ciliary membrane, as described in detail in the following sections. Therefore, the initial events of transduction take place in the cilia.

FIGURE 8.3
Spatial distribution of sensitivity to an odorant stimulus. An isolated ORC was stimulated under voltage-clamp condition by a brief odorant pulse (50 ms duration indicated by open arrows) from a glass pipette (tip diameter, ~1 μm) placed at numbered (more...)
8.3.2. Signal Amplification
Since the signal coming from the outside could be very small, receptor cells must have amplification mechanisms. In the olfactory cilia, two different types of signal amplification are present in the transduction cascade: (a) molar amplification and (b) nonlinear amplification. The former increases the number of active molecules, while the latter changes the input-output relation in a nonlinear way with a high cooperativity. The relation between odorant dose and peak receptor current is generally well fit by a Hill equation: I/Imax = CnH/(CnH + K1/2nH), where Imax is the maximal current, C is the concentration of odorant, K1/2 is the half-maximal effective concentration, and nH is the Hill coefficient, which corresponds to the cooperativity of the system. As shown in Figure 8.4B, in ORCs the Hill coefficient can reach values higher than 6. With such a nonlinear amplification, only a slight change in the concentration of odorant molecules produces a large change in the response.

FIGURE 8.4
(A) Schematic diagram showing the molecular elements of olfactory transduction. (B) Dose response to odorant stimulation in isolated newt ORCs under voltage-clamp condition (holding potential −50 mV). (C) Peak amplitudes of responses obtained (more...)
8.3.3. Adaptation or Desensitization
Olfactory sensation gradually decreases during long or repeated exposures to odorant stimuli (see e.g., Getchell and Shepherd 1978). This phenomenon involves many processes along the entire olfactory pathway, but it begins in the cilia of ORCs. Indeed, during application of a prolonged odorant stimulus, the receptor current decreases with time, despite the continued presence of the stimulus, as shown in Figure 8.5A (Kurahashi and Shibuya 1990; Firestein et al. 1990; Menini 1995; Reisert and Matthews 1999; Zufall et al. 1991). On the other hand, when two brief odorant pulses are delivered within a short interval, the amplitude of the response to the second pulse is reduced (Kurahashi and Shibuya 1990; Kurahashi and Menini 1997). At the time of the second odorant stimulus, the cell is in an adapted state, the first (conditioning) pulse having desensitized the neuron to subsequent stimuli. The desensitization is greater with shorter interstimulus intervals as shown in Figure 8.5B (Kurahashi and Shibuya 1990; Kurahashi and Menini 1997; Takeuchi et al. 2003), and the current amplitude gradually recovers to the initial value, increasing the interval between odorant pulses.
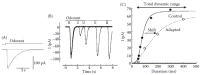
FIGURE 8.5
Adaptation in olfactory receptor cells. (A) Adaptation during prolonged odorant stimulation. An isolated ORC was stimulated under voltage-clamp condition by a long odorant pulse. The transduction current declines despite the continuous presence of the (more...)
Sensory adaptation is not merely a reduction in response amplitude, but its physiological role involves the adjustment of the response to allow a cell to work over a broad range of stimuli (for review, see Torre et al. 1995). Indeed, in odorant adaptation to repetitive stimuli there is a shift of the dynamic range (i.e., the range of stimulus concentrations over which the OSN is able to respond) toward higher odorant concentrations compared with the control state (Kurahashi and Menini 1997; Boccaccio et al. 2006; Reisert and Matthews 1999). In the adapted state, a stronger stimulus is required to produce a half-maximal response with respect to control condition (Figure 8.5C). During a prolonged exposure to an odorant, adaptation is expected to continuously reset the neuron to discriminate higher odorant concentrations without saturating the transduction process.
8.3.4. Masking
In human history, odorants have been widely used to induce pleasant smells. At the same time, some odorants (like flavors and fragrances) have also been used for masking unpleasant smells. In particular, a wide-spectrum odorant suppression has been employed in the smell-masking industries, such as the usages of spices and the development of perfumes. It has been suggested that the masking effect begins at the receptor cell level. The response from the single ORC is suppressed by the stimulant itself or by additional odorants (Kurahashi et al. 1994). The significance and the mechanisms of the olfactory suppression will be explained in Section 8.5.4.
8.4. MOLECULAR MECHANISMS OF OLFACTORY TRANSDUCTION
8.4.1. Odorant Receptors and Cyclic Adenosine Monophosphate (cAMP) Formation
A large majority of ORCs express a member of the odorant receptor family discovered by Buck and Axel (1991). Odorant receptors belong to the superfamily of G-protein-coupled receptors and have the same general structure with seven hydrophobic membrane-spanning regions, but they differ in their amino acid sequence, especially in transmembrane domain III, IV, and V, suggesting that these parts are responsible for the discrimination of odorant species (Mombaerts 2004). In the mouse, there are about 1000 genes encoding different types of odorant receptors. Each ORC expresses only one type of odorant receptor gene in its ciliary membrane. The reader is referred to Chapter 7 of this book for a detailed discussion about odorant receptors.
A single ORC responds to many types odorants and, on the other hand, a single type of odorant can stimulate several types of ORCs expressing different odorant receptors (Firestein et al. 1993). The random distribution of odorant receptors and their broad selectivity are characteristics of olfactory coding at the level of the olfactory epithelium.
As shown in Figure 8.6, the ligand-bound receptor activates an olfactory-specific excitatory G-protein, Gαolf (Jones and Reed 1989), whose structure is similar to that of other types of G-proteins. It consists of three subunits, α,β, and γ, at the cytoplasmic surface of the ciliary membrane. By homology with other G-proteins, it is likely that after the odorant binds to a receptor, Gαolf exchanges guanosine 5′-diphosphate (GDP) for guanosine 5′-triphosphate (GTP), and the GTP-bound Gαolf subunit dissociates from the β and γ subunits and activates AC type III (Bakalyar and Reed 1990). This is an integral membrane protein with 12 transmembrane domains, both C- and N-terminals at the intracellular side, and the catalytic domain located between transmembrane domain 6 and 7. The catalytic region of AC converts adenosine triphosphate (ATP) into cAMP, a molecule that plays a fundamental role in olfaction as a second messenger and mediates signal transduction for a wide variety of odorants (Brunet et al. 1996; Lowe et al. 1989; Takeuchi and Kurahashi 2003). cAMP is a small and water-soluble molecule, with a molecular weight of 329. The diffusion coefficient of cAMP in frog olfactory cilia was estimated to be 2.7 x 10−6 cm2 s−1 (Chen et al. 1999), which provides an average one-dimensional diffusion speed of approximately 20 μm/s. If cAMP is generated at the tip of the olfactory cilia, it could diffuse through the ciliary cytoplasm to the base of the cilia during the odorant response, which typically lasts a couple of seconds (Figure 8.4B). In the olfactory cilia, the actual spread of cAMP seems to be limited (Takeuchi and Kurahashi 2008). This is probably due to several factors, including the binding of cAMP to CNG channels and the activity of the phosphodiesterase PDE1C2, expressed almost exclusively in the cilia (Borisy et al. 1992; Yan et al. 1995).
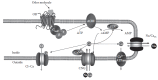
FIGURE 8.6
Molecular mechanisms of olfactory transduction. The binding of odorant molecules to an odor-ant receptor (OR) induces the G-protein-mediated activation of adenylyl cyclase. cAMP directly gates CNG channels, generating a depolarizing influx of Na+ and (more...)
8.4.2. Cyclic Nucleotide-Gated Channels
CNG channels are the mediators of the chemoelectrical energy conversion in olfactory cilia. Indeed, information about odorant molecules is first transmitted as chemical information and then, at the level of the CNG channel, is converted into an electrical signal by the activation of ion fluxes across the plasma membrane. This membrane protein consists of four subunits containing two CNGA2, one CNGA4, and one CNGBlb (Zheng and Zagotta 2004). Each subunit has six transmembrane domains (S1-S6), and a pore region between S5 and S6 domains. A cyclic nucleotide-binding site is located near the C-terminal at the cytoplasmic side in each subunit (Dhallan et al. 1990), for a total of four binding sites per each CNG channel. The cAMP binding and channel activation show an allosteric effect, by which the CNG channel displays cooperativity (Hill coefficient of 2). Recently, along this line, Nache et al. (2005) and Biskup et al. (2007) demonstrated that in homomeric CNGA2 channels the binding of the second cAMP molecule brings the channel almost to its maximum open probability of about 0.7–0.8 (Kurahashi and Kaneko 1993; Kleene 1997; Larsson et al. 1997; Reisert et al. 2003).
It is significant to note that CNG channels are permeable to monovalent ions, such as Na+ and K+, and also to Ca2+ (PCa/PNa = 6.5; Kurahashi and Shibuya 1990). The fraction of current carried by Ca2+ was estimated to be 0.4 in heterologously expressed rat CNG channels in the presence of 2 mM of extracellular Ca2+ at −70 mV (Dzeja et al. 1999). This Ca2+ influx results in an increase of Ca2+ in the intraciliary medium and this has important roles in olfactory transduction.
The unitary conductance of the single CNG channel is about 30 pS in the absence of divalent cations, but this value becomes smaller when the external solution contains Ca2+ or Mg2+ ions (Zufall and Firestein 1993). Indeed, Ca2+ and Mg2+ entry at negative potentials produces an open channel block causing an increase in flickering activity of the channel. This temporary block reduces the current carried by all cations, producing a very small, single channel conductance (~ 1.5 pS, Zufall and Firestein 1993; 0.56 pS, Kleene 1997). A small, single channel conductance plays a relevant physiological role, since, by using a large number of tiny events, the integrated current has a high signal-to-noise ratio (Kurahashi and Kaneko 1991; for review see Kleene 2008).
The channel density in the cilium has been estimated by electrophysiological methods with widely differing results: 1750 channels/μm2 in the toad (Kurahashi and Kaneko 1993), 67–202 channels/μm2 in the frog (Kleene 1994; Larsson et al. 1997), and 8 channels/μm2 at the dendritic knob/cilia in the rat (Reisert et al. 2003).
8.4.3. Calcium-Activated Chloride Channels
Ca2+ ions entering the cilia through CNG channels play crucial roles in olfactory transduction; namely the activation of Cl(Ca) channels and the regulation of adaptation (for review, see Matthews and Reisert 2003; Menini 1999). Lateral (longitudinal) diffusion of Ca2+ seems to be restricted, because the responses to double pulse local laser stimuli applied to different parts along the cilium are independent, and do not show Ca2+-dependent adaptation (Takeuchi and Kurahashi 2008; for adaptation see Section 8.5.2). Within the cilia, Ca2+ ions are buffered by Cl(Ca) channels, calmodulin (CaM), and some unidentified Ca2+-binding proteins (see, e.g., Uebi et al. 2007).
Olfactory signal transduction has the peculiarity that electrical excitation is generated by the activation of two different types of ion channels: CNG and Cl(Ca) channels (Figure 8.6). Cl(Ca) channels are present in the ciliary membrane and are activated by a rise in ciliary Ca2+ concentration (Kleene 1993; Kleene and Gesteland 1991; Lowe and Gold 1993; Kurahashi and Yau 1993). Moreover, as shown in Table 8.1 and discussed in Section 8.4.4, ORCs maintain an elevated intracellular Cl− concentration that is in the same range as the Cl− concentration present in the mucus at the external side of the cilia (Kaneko et al. 2001; Reuter et al. 1998). Therefore, in physiological conditions, the opening of Cl(Ca) channels in the ciliary membrane causes an efflux of Cl− ions from the cilia, corresponding to an inward current that contributes further to the depolarization of these neurons.
It has been suggested that the presence of a pair of cationic and anionic currents in the depolarizing response to odorants is useful because the Cl− current produces a large amplification of the primary cationic CNG current (Lowe and Gold 1993) and the amplified signal has a higher signal-to-noise ratio than the primary signal (Kleene 1997). Therefore, in olfactory transduction, the secondary Cl(Ca) channels ensure a high-gain and low-noise amplification of the primary CNG current, contributing up to 90% of the total odorant-induced current (Boccaccio and Menini 2007; for reviews, see Kleene 2008; Frings et al. 2000, 2009).
The functional properties of the Cl(Ca) conductance have been investigated under various conditions with a variety of electrophysiological techniques, each revealing important information about the channel properties. The electrical activity in response to odorants, which can be recorded at the surface of the olfactory epithelium as a negative electrical field potential, the electro-olfactogram (EOG) (Ottoson 1955; Scott and Scott-Johnson 2002) has been shown to be primarily caused by the depolarizing action of Cl− current, since more than 80% of the response can be blocked by niflumic acid (NFA), a blocker for Cl− channels (Nickell et al. 2006). The large contribution of the Cl− conductance to the transduction current is confirmed by experiments in isolated cells obtained with the suction pipette or in the whole-cell voltage clamp configuration, showing that the fraction of the current carried by Cl− can be up to 90% in mice (Boccaccio and Menini 2007; Reisert et al. 2005). The activation of the conductance by Ca2+ has been carefully investigated in inside-out excised membrane patches from the knob/cilia of mouse ORCs. Studies in the mouse (Reisert et al. 2005; Pifferi et al. 2006b) have shown that the dose-response relation is well fit by the Hill equation with half maximal activation between 2.2 and 4.7 μM Ca2+, and Hill coefficient between 2.0 and 2.8. Moreover, this conductance shows a Ca2+-dependent inactivation, which is reversible after a few seconds removal of Ca2+ (Reisert et al. 2003, 2005), but also an irreversible run-down indicating that some modulatory component of the channel may be lost after the excision of the membrane (Reisert et al. 2003, 2005). The native olfactory Cl(Ca) channel is apparently not affected by Ca2+-CaM (Reisert et al. 2003; Kleene, 1999) and, at present, no modulators of the channel activity are known.
The current conducted by a single olfactory Cl− channel is so small that single-channel studies have not been possible. By noise analysis of macroscopic currents, the unit conductance was estimated to be 1.3 pS in the rat (Reisert et al. 2003) and 1.6 pS in the mouse (Pifferi et al. 2006b), with a channel’s maximum open probability of .97. The combination of a very small single channel conductance, together with a high maximum open probability, allows a high amplification of the primary current without an increase of noise (Kleene 1997).
Specific blockers for Cl(Ca) with high binding affinity are not available, but some commonly used extracellular blockers of Cl(Ca) include NFA and 4-acetamido-4′-isothiocyanato-stilben-2,2′-disulfonate (SITS) (Lowe and Gold 1993; Kleene 1993; Kurahashi and Yau 1993; for review, see Frings et al. 2000).
The molecular identity of Cl(Ca) channels is still elusive, although some proteins have been proposed as possible candidates for being a molecular component of the conductance, including bestrophin-2 (mBest2) and TMEM16B (AN02) (Pifferi et al. 2006b; Pifferi et al. 2009; Stephan et al. 2009). Pifferi et al. (2006b) showed that mBest2 mRNA is expressed in ORCs, and that the protein is expressed in the cilia of ORCs, where it colocalizes with CNGA2, the principal subunit of the olfactory CNG channel responsible for the primary transduction current (Pifferi et al. 2006b). Moreover, the functional properties of the current induced by heterologous expression of mBest2 and those of the native Cl(Ca) channels from the dendritic knob/cilia of mouse ORCs have many similarities, but also some differences (Pifferi et al. 2006b). Indeed, the two currents have the same anion permeability sequence, small estimated single-channel conductances, and the same side-specific blockage by two Cl− channel blockers (NFA and SITS). The most significant difference between the two currents is the sensitivity to intracellular Ca2+. In fact, currents are half-maximal at a Ca2+ concentration of 0.4 μM for mBest2, whereas native currents require a higher Ca2+ concentration, 4.7 μM. Very recently, it has been proposed that the anoctamin/TMEM16 family of membrane proteins are Cl(Ca) channels (Caputo et al. 2008; Yang et al. 2008; Schroeder et al. 2008). Interestingly, a previous study showed that Tmeml6b is expressed in the mature sensory neurons of the olfactory epithelium by in situ hybridization (Yu et al. 2005). Proteomic screenings of ciliary membranes recently revealed that TMEM16B is a prominent protein in the olfactory cilia (Mayer et al. 2009; Stephan et al. 2009). Moreover, Stephan et al. (2009) showed that the fusion protein TMEM16B-EGFP localized to the cilia when expressed in vivo using an adenoviral vector. A comparison of the properties of TMEM16B-induced currents upon heterologous expression in a mammalian cell line with those of native Cl(Ca) in the sensory neurons of the olfactory epithelium indicates that the two channels are remarkably similar (Pifferi et al. 2009; Stephen et al. 2009). At present, antibodies against Tmeml6b are not available, therefore, it is not possible to establish whether the protein is expressed in the cilia. Future studies combining a multidisciplinary approach from genetic, molecular biology and electrophysiology will be necessary to reveal the involvement of mBest2, TMEM16B, and other candidates in the olfactory Cl(Ca).
8.4.4. Ion Homeostasis
Since electrical excitation is due to ion fluxes across the cell membrane, the ion homeostasis is very important in olfactory signal transduction. The concentration of Ca2+ inside the cilia increases during odorant stimulation and is restored to basal levels by two major mechanisms: extrusion of Ca2+ by Na7Ca2+ exchanger and Ca2+-ATPase (Figure 8.6). The indication of the presence of a Na7Ca2+ exchanger in the dendrite, dendritic knob, and possibly in the cilia, was initially proposed by Jung et al. (1994) and Noe et al. (1997), and was confirmed physiologically by Reisert and Matthews (1998) on the basis of ion substitution experiments. Indeed, it is well known that the Na+ driving force establishes Ca2+ extrusion by the Na7Ca2+ exchanger and that the substitution of Na+ bathing the cilia with another cation, such as choline+ or Li+, known for inhibiting the exchanger, should inhibit Ca2+ efflux. Reisert and Matthews (1998) measured the time course of the Cl(Ca) current component with the suction (loose seal) electrode. Since the Cl(Ca) current is activated by the presence of cytoplasmic Ca2+, the time course of Cl(Ca) represents an index of the intracellular Ca2+ concentration. They observed that the current response was greatly prolonged in the absence of external Na+, which indicates that Ca2+ was not extruded, suggesting that the Na7Ca2+ exchanger is the main mechanism that returns cytoplasmic Ca2+ concentration to basal levels after stimulation. Furthermore, Reisert et al. (2003) showed that the Na+-dependent Ca2+ extrusion can also be detected in the excised patch membrane obtained from the apical dendrite. Antolin and Matthews (2007) further investigated the Na+-dependence of the exchanger in the frog and found that the rate of Ca2+ extrusion was only modestly affected by extracellular Na+ until its concentration was 30% of its value in Ringer solution, suggesting that Ca2+ extrusion from the cilia would be expected to be only marginally affected by modest changes in mucus Na+ concentration.
The olfactory Na7Ca2+ exchanger seems to be independent of potassium ion (Reisert et al. 2003), different from the Na7Ca2+ exchanger of rod photoreceptor cells, in which the potassium ion is cotransported. However, Pyrski et al. (2007) reported that ORCs also express the gene encoding for the potassium-dependent Na7Ca2+ exchangers (NKCX1–3), although at present there is no functional evidence that they are involved in Ca2+ clearance during olfactory transduction.
Another major mechanism that reduces the intraciliary Ca2+ concentration is the activity of Ca2+-ATPase. Several isoforms of Ca2+-ATPase have been localized to the cilia by immunohistochemistry (Castillo et al. 2007; Weeraratne et al. 2006). Moreover, Castillo et al. (2007) showed that, in rat and toad ORCs, the current relaxation time-course of the cAMP-induced response (induced by the photolysis of cytoplasmic caged cAMP) is prolonged in the absence of intracellular ATP and by blocking the Ca2+-ATPase by carboxyeosin. These results may indicate that Ca2+-ATPase is also involved in Ca2+ extrusion from the cilia.
In the nervous system, though openings of Cl− channels usually produce inhibitory responses in neurons, as exemplified by the GABAergic and glycinergic synapses, in ORC the CF current is excitatory and amplifies the primary transduction current. To generate an excitatory CF current, ORCs have CI uptake systems to maintain a high intracellular Cl− concentration. Using 2-photon ion imaging, Kaneko et al. (2004) reported that the uptake mechanism of Cl− resides in the apical membrane of ORCs, most probably within the ciliary membrane or in the membrane of the dendritic knobs. Moreover, Reisert et al. (2005) showed that a Na7K+/2CF cotransporter (NKCCl) greatly contributes to Cl− homeostasis in the ORC, since ORCs from mice lacking NKCCl are not able to maintain the high intracellular Cl− concentration necessary to sustain the excitatory Cl(Ca) current. By immunohistochemistry, NKCCl was localized in the soma and in the dendrite, but not in the cilia (Reisert et al. 2005). It is likely that additional Cl− accumulation systems are present. Indeed, Nickell et al. (2007) showed that multiple chloride transporter systems are expressed in the mouse olfactory epithelium. At present, the mechanisms for the Cl− homeostasis in the ORC and its cilia are still unclear.
8.5. INTERACTIONS BETWEEN CILIARY ION CHANNELS AND CYTOSOLIC MESSENGERS
Olfactory transduction occurs in the olfactory cilia by means of the molecular elements described above. These molecules with their own integrative properties shape the outline of the sensory characteristics, and their different functional roles are illustrated in Figure 8.7. Odorant molecules bind to odorant receptors and the binding switches on the transduction cascade. It has been indicated that the odorant dwell-time on the receptor is at most of the order of 1 ms (Bhandawat et al. 2005), but the active signaling time is extended at the level of the G-protein and AC to several hundreds of milliseconds at upstream points to AC (Takeuchi and Kurahashi 2002). Furthermore, even after the inactivation of AC, the signal remains as cytoplasmic cAMP, cAMP-CNG complex, intraciliary Ca2+, and Ca-Cl(Ca) complex. Such a time extension produces a molar amplification. In fact, the opening of only a 0.01 pA single CNG channel for 1 ms allows the entry of 70 Na+ ions in the cilia. At the CNG and Cl(Ca) channels, the cooperativity is increased to 6, as illustrated in Figure 8.4B, showing a nonlinear amplification. Because of this, a single ORC can detect a very small change in odorant concentration and the response becomes almost all-or-nothing.
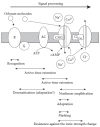
FIGURE 8.7
Functional roles of molecular elements of olfactory transduction. Schematic diagram showing the involvement of the different molecular elements in the shaping of the characteristic features of olfactory transduction.
The combination of CNG and Cl(Ca) channels carrying ions in opposite directions has the important physiological advantage of making the olfactory response resistant against possible changes in the ionic composition surrounding the cilia (Kleene and Pun 1996).
Short-term adaptation is regulated by the Ca2+ feedback to the CNG channel, and also at some point, not yet identified, before activation of AC (Takeuchi and Kurahashi 2002). Finally, olfactory masking is regulated at the CNG channel level by the direct odorant suppression of the channel (Chen et al. 2006), whereas the Cl(Ca) channel is not involved in this process (Takeuchi et al. 2009).
8.5.1. Molecular Mechanisms of Odorant Adaptation in the Cilia
As described in Section 8.3.3, the ORC itself has the ability to adapt. This sensory adaptation occurs in the ciliary membrane and it depends on Ca2+ entry through CNG channels (Kurahashi and Shibuya 1990). Kurahashi and Menini (1997) investigated the localization of the principal molecular mechanism for adaptation in the olfactory transduction process. To determine whether the response reduction in the adapted state was attributable to a reduction in the cAMP production or was instead due to other processes occurring after the production of cAMP, CNG channels in intact neurons were directly activated by flash photolysis of caged cAMP. The ciliary cytoplasm was loaded with caged cAMP through diffusion from a patch pipette, and application of ultraviolet flashes to the cilia caused the photorelease of various cAMP concentrations. Therefore, cAMP-gated channels can be directly activated, bypassing the early stages of odorant transduction (i.e., receptor activation and G-protein and adenylate cyclase signaling). cAMP and odorant-induced responses were found to have similar adaptation properties, indicating that the entire adaptation process takes place after the production of cAMP (Kurahashi and Menini 1997; Menini 1999; but see also Takeuchi and Kurahashi 2002).
By using a hydrolysis-resistant caged cAMP analog, caged 8Br-cAMP, Boccaccio et al. (2006) have shown that the hydrolysis of cAMP by PDE is not involved in adaptation. Furthermore, Cl(Ca) channels have been shown to be unrelated to olfactory adaptation (Boccaccio et al. 2006; Kurahashi and Menini 1997).
Several data show that CNG channels are modulated by a Ca2+-dependent feedback. In native CNG channels, it has been demonstrated that the addition of micromolar concentrations of intracellular Ca2+ is able to decrease the channel sensitivity to cAMP, probably by activating a Ca2+-responsive endogenous factor already preassociated with the channel (Kramer and Siegelbaum 1992; Lynch and Lindemann 1994; Balasubramanian et al. 1996; Bradley et al. 2004). Bradley et al. (2004) have shown that Ca2+-free CaM, called apocalmodulin, is able to bind to the heterologously expressed heteromeric olfactory CNG channels even in the absence of Ca2+. Moreover, when Ca2+ concentration rises above 100 nM, Ca2+ can rapidly modulate the CNG channel sensitivity by binding directly to the preassociated CaM. Furthermore, it was suggested that in native channels also, the preassociated endogenous factor could be apocalmodulin, although a direct demonstration is still missing (Bradley et al. 2004). Since Ca2+ enters into the olfactory cilia through the CNG channel itself, the preassociated Ca2+ responsive factor could provide a very fast feedback modulation at the channel level.
Early works (Chen and Yau 1994; Liu et al. 1994; Varnum and Zagotta 1997; Grunwald et al. 1999; Zheng et al. 2003) identified in the N-terminus of CNGA2 a classic basic amphiphilic α-helix (Baa) motif with high affinity for Ca2+-CaM and showed that the sensitivity to cAMP of heterologously expressed homomeric CNGA2 channels was decreased by the binding of Ca2+-CaM to the Baa motif (Figure 8.8A). However, in recent years, there has been considerable progress in elucidating the molecular events producing modulation of the native channels and it has been shown that the Baa motif of CNGA2 does not play any role in Ca2+-CaM modulation of heteromeric channels. On the contrary, by comparing properties of native channels with heterologously expressed heteromeric channels, the modulatory subunits CNGA4 and CNGBlb have been shown to be responsible for the physiological modulation of Ca2+-CaM (Bradley et al. 2001, 2004). Munger et al. (2001), in excised patches containing native heteromeric olfactory CNG channels, measured a fast current inhibition on the addition of Ca2+-CaM, which persisted for several seconds also after CaM was removed in Ca2+-free solution (Figure 8.8B). In contrast, homomeric CNGA2 showed a slower onset of inhibition by Ca2+-CaM and a faster recovery, suggesting that CNGB1 and CNGA4 mediated the physiological relevant modulation of the channel (Bradley et al. 2001).
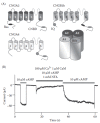
FIGURE 8.8
CNG modulation and adaptation in ORCs. (A) Topological model and assembly of subunits of the olfactory CNG channel. Each transmembrane domain is indicated by a number, the pore loop is located between 5 and 6. The cyclic nucleotide-binding site (CNBD) (more...)
Indeed, the modulatory subunits also have CaM-binding sites: CNGA4 has an IQ-type CaM-binding site located at the C-terminal region, while CNGBlb has a similar IQ-type site located at the N-terminal region and a Baa motif in the C-terminal region (Figure 8.8A). It has been shown that the IQ-type sites are necessary and sufficient for Ca2+-CaM channel inhibition, whereas the Baa-type site is not necessary (Bradley et al. 2001, 2004). Moreover, ORCs from knockout mice for CNGA4 showed a reduced adaptation both after prolonged odorant stimulation and with double pulse protocol (Munger et al. 2001). However, these data have been partially challenged by a subsequent report showing that the lack of CNGA4 or CNGB1 greatly reduced the sensitivity of the channel to cAMP and also impaired the trafficking of subunits to olfactory cilia (Michalakis et al. 2006). Indeed, Song et al. (2008) produced mice with CNG channels lacking the IQ-type CaM-binding site in the CNGB1 subunit, which should make the channels insensitive to CaM modulation, but with normal trafficking and cAMP sensitivity. They reported that adaptation to double stimulation was not affected by the binding site deletion, suggesting that CaM did not play a pivotal role in this process. However, in this mouse line, the response to odorants shows a longer termination both after brief and long-lasting odorant stimulations, pointing out the role of Ca2+-CaM modulation in shaping the termination of the odorant response (Song et al. 2008).
These results could also suggest that CaM is not the Ca2+-responsive factor that is coassembled with the CNG channel (Bradley et al. 2001). Other experimental evidence argue against this hypothesis, in particular the endogenous factor appears to bind to the CNG channels in a very stable manner, being washed away only after intense rinsing in Ca2+-free solution (Kramer and Siegelbaum 1992; Kleene 1999; Bradley et al. 2004). However, it is also possible to speculate that the binding of “native” CaM is more stable because the channel or the CaM itself undergoes post-transductional modifications that change the properties of the interaction. On the other hand, it cannot be excluded that other proteins, in addition to CaM, contribute to the Ca2+-mediated modulation of olfactory CNG channels (Pifferi et al. 2006a).
8.5.2. Spatial Arrangements of Transduction Channels
To understand the signal transmission process between CNG and Cl(Ca) channels, including nonlinear processes mediated by cytoplasmic Ca2+, it is important to know their spatial arrangements along the cilium. Localization of CNG channels along the cilium has been investigated both with electrophysiological and with electron microscopy immunogold, while for the distribution of Cl(Ca) channels, only electrophysiological techniques have been used, because the molecular identity of these channels is still unknown.
8.5.2.1. Distribution of Cyclic Nucleotide-Gated Channels
Nakamura and Gold (1987) performed the first experiments showing that CNG channels are present in the ciliary membrane, by directly activating the channels with cAMP in inside-out excised patches from the toad ciliary membrane. Kurahashi (1990) demonstrated that these channels are mainly localized in the cilia in whole-cell experiments, allowing cAMP to diffuse from the patch pipette into the cell interior. He observed that the response became larger and its latency shorter when the patch pipette was situated at the apical dendrite than when it was positioned at the soma. When examined with excised inside-out patches, the density of CNG channels in the newt was 1000 channels/μm2 at the cilia, while in the dendrosomatic membrane there were only a few channels/μm2 (Kurahashi and Kaneko 1991). This polarized distribution of CNG channels is consistent with the observation that odorant sensitivities are high at the cilia (Figure 8.3). The density of CNG channels has also been measured in the frog using a detached cilia preparation (Larsson et al. 1997). The current fluctuations accompanied with the activation of CNG channels were analyzed in non-space-clamped cilia and the channel density was estimated to be 70 channels/μm2.
The distribution of CNG channels along the cilia was investigated by electron microscopy immunogold against CNGA2 and with electrophysiological techniques. Electron microscopy showed that CNGA2 was predominantly localized at the tip of the cilia (Matsuzaki et al. 1999). Flannery et al. (2006) used the detached inside-out ciliary preparation and a cAMP diffusion model, and found that the proximal segment, which in the frog is the first 20% of the cilium, appears to express a small fraction of the CNG channels, whereas the distal segment contains their majority, mostly clustered in one region. Furthermore, Takeuchi and Kurahashi (2008) used submicron local laser spot to photolyze cytoplasmic caged cAMP (see Figure 8.1B), and examined the localization of the cAMP-induced current. Since a response was obtained everywhere along the single cilium, CNG channels were considered to be broadly distributed along the cilium. Local responses were therefore amplified by the high density of CNG channels, eliciting >100 pA current with a stimulus illuminating only 1 μm length cilium (Takeuchi and Kurahashi 2008). This high current value is surprising if we consider that, given the very high input resistance of ORCs, 1 pA is sufficient to generate action potentials (Lynch and Barry 1989) that transmit the olfactory information to the brain.
8.5.2.2. Distribution of CI(Ca) Channels
Kleene and Gesteland (1991) first discovered the presence of a large Cl(Ca) current in the detached cilia preparation. With noise analysis, Larsson et al. (1997) showed that the density of Cl(Ca) channels in the cilia of frog ORCs is almost comparable to that of the CNG channels (70 channels/μm2). In contrast, Reisert et al. (2003) reported that in rat ORCs the density of Cl(Ca) channels at the knob/cilia was about eightfold bigger than that of the CNG channels (62 vs 8 channels/μm2).
Takeuchi et al. (2009) employed laser photolysis of intraciliary caged Ca2+, and investigated the localization of Cl(Ca) along the cilia. The channel distribution was broad along the cilia, displaying a spatial pattern similar to that of CNG channels. The broad distribution of Cl(Ca) channels provides evidence that all molecular elements involving electrical excitation are distributed along the cilia. This is consistent with the theoretical estimation by Reisert et al. (2003) who suggested that CNG and Cl(Ca) channels are closely arranged on the plasma membrane, although they do not constitute transduction complexes. If the CNG and Cl(Ca) were to separate, internal diffusion of Ca2+ would be necessary to activate Cl(Ca) channels, giving rise to a more complex transmission of information between these sequentially chained ion channels. The functions of Cl(Ca) channel are, therefore, homogeneous along the cilium, similar to the CNG channels.
8.5.3. Linear Spatial Summation of Local Responses: Hindered Diffusion of Cytoplasmic Factors?
Takeuchi and Kurahashi (2008) showed that the cAMP responses induced by submicron local photolysis (Figure 8.1B) were independent within the cilium, especially when the response amplitude was small. Even within a 2 μm distance, double laser stimuli released within a short interval produced the same response amplitudes. This may indicate that the odorant-binding signal sums linearly, independent of the stimulus position and timing. Such linear summation of the local responses may suggest that the diffusion of cytoplasmic factors (cAMP and Ca2+) is hindered, when a limited number of molecules are produced locally. If cAMP and/or Ca2+ could travel far from the site of photolysis, the adjacent response must be reduced by Ca2+-dependent adaptation. The diffusion of cytoplasmic factors within the thin cylindrical structure is obviously determined not only by their intrinsic diffusion properties, but also by buffering inside the cilium, and by extrusion/degradation systems. Furthermore, it should be noted that, in the ciliary nanostructure, the surface to volume ratio is extremely high and, therefore, soluble molecules have a high probability of interacting with molecules bound at the cytoplasmic membrane surface. Takeuchi and Kurahashi (2005) estimated that the maximum cytoplasmic cAMP level was of the order of 100 μM, which is equivalent to ~500 molecules/μm-length (calculated from 0.1 μm diameter of the cilium). Since the density of the CNG channel is 300 channels/μm-length cilia (Kurahashi and Kaneko 1991), and each CNG channel has four cAMP binding sites (see e.g., Zheng and Zagotta 2004), the total number of cAMP binding sites in 1 μm-length cilia is 1200. Therefore, the number of cAMP binding sites (1200) available is higher than the number of cAMP molecules produced by the odorant (500). Single CNG channels show open (bursting) events with a mean open time of ~100 ms, which is likely to represent the mean lifetime of the cAMP-CNG complex (Kurahashi and Kaneko 1993). Therefore, the CNG channels will initially trap most of the produced cAMP molecules with a very efficient surface to volume ratio, while some of them will be hydrolyzed by PDE. These processes may, at least in part, explain the outline of the hindered diffusion for the limited number of local cAMP molecules.
Because of the described properties of spatial linearity, ORC responses to small stimuli are additive, which increases the quantum efficiency of signal detection. At strong odorant stimulation, the length constant of the cilia becomes short and the total current reaches saturation before the CNG and Cl(Ca) are fully activated. Due to such saturation, the ciliary membrane does not pass the ions at a distal area. This may help avoid Cl− depletion in the cilia.
8.5.4. Cyclic Nucleotide-Gated Channels: An Initial Point For Signal Amplification and Site of Signal Modulation
As described in Section 8.5.1, CNG channels are involved in olfactory adaptation. A recent study indicates that CNG channels are also involved in the molecular mechanisms underlying olfactory masking. Indeed, Takeuchi et al. (2009) showed that not only can several types of odorants reduce the current through the CNG channel, but that the resulting odorant spectra show a positive correlation with olfactory masking measured in humans. Thus, at the olfactory cilia, both adaptation and masking are regulated at the level of CNG channels, although the mechanisms are different: negative feedback regulation in the case of adaptation and direct block by some odorants for masking. Interestingly, the Cl(Ca) channel is not directly modulated in both phenomena (Boccaccio et al. 2006; Takeuchi et al. 2009), although a reduction in the CNG current causes a reduction of Ca2+ influx, which, in turn, reduces the amplitude of Cl(Ca) current.
It has been shown that the odorant- and cAMP-induced responses show similar cooperativities (see Figure 8.4C and E; Takeuchi and Kurahashi, 2005), indicating that a nonlinear amplification is established at a downstream point from cAMP production, due to the involvement of both CNG and Cl(Ca) channels. Indeed, each channel is activated in a cooperative way and their sequential activation gives origin to a highly nonlinear amplification. Therefore, it is sufficient to modulate the primary CNG channel to affect adaptation and masking in a nonlinear way. In this respect, the modulation on CNG channels is expanded as an efficient effect at the transmission process (Figure 8.7). Furthermore, by directly modulating CNG instead of Cl(Ca) channels, Ca2+ influx can be economically regulated. Since both channels are distributed evenly along the entire cilia, both signal generation and modulation are homogeneously regulated.
8.6. CONCLUDING REMARKS
In this chapter, we have reviewed the basic principles of olfactory transduction in vertebrate olfactory cilia with a special emphasis on the functional role of CNG and Cl(Ca) channels and their interaction with intracellular messengers. Although several components and modulators of the transduction cascade have been identified at the molecular level, many others, including Cl(Ca) channels, some ion exchangers, and Ca2+-binding proteins, remain to be identified. Their discovery will allow a better understanding of the transduction process occurring in our thin olfactory cilia.
ACKNOWLEDGMENTS
We thank Hiroko Takeuchi for interesting discussions. This work was supported by grants from: JSPS (T.K.), the Italian Ministry of Research (MIUR), and the Italian Institute of Technology (A.M.).
REFERENCES
- Antolin S., Matthews H.R. The effect of external sodium concentration on sodium-calcium exchange in frog olfactory receptor cells. J Physiol. 2007;581:495–503. [PMC free article: PMC2075203] [PubMed: 17379630]
- Bakalyar H.A., Reed R.R. Identification of a specialized adenylyl cyclase that may mediate odorant detection. Science. 1990;250:1403–6. [PubMed: 2255909]
- Balasubramanian S., Lynch J.W., Barry P.H. Calcium-dependent modulation of the agonist affinity of the mammalian olfactory cyclic nucleotide-gated channel by calmodulin and a novel endogenous factor. J Membr Biol. 1996;152:13–23. [PubMed: 8660407]
- Bhandawat V., Reisert J., Yau K.W. Elementary response of olfactory receptor neurons to odorants. Science. 2005;308:1931–34. [PMC free article: PMC2957801] [PubMed: 15976304]
- Biskup C., Kusch J., Schulz E., Nache V., Schwede F., Lehmann F., Hagen V., Benndorf K. Relating ligand binding to activation gating in CNGA2 channels. Nature. 2007;446:440–43. [PubMed: 17322905]
- Boccaccio A., Lagostena L., Hagen V., Menini A. Fast adaptation in mouse olfactory sensory neurons does not require the activity of phosphodiesterase. J Gen Physiol. 2006;128:171–84. [PMC free article: PMC2151529] [PubMed: 16880265]
- Boccaccio A., Menini A. Temporal development of cyclic nucleotide-gated and Ca2+-activated Cl– currents in isolated mouse olfactory sensory neurons. J Neurophysiol. 2007;98:153–60. [PubMed: 17460108]
- Borisy F.F., Ronnett G.V., Cunningham A.M., Juilfs D., Beavo J., Snyder S.H. Calcium/calmodulin-activated phosphodiesterase expressed in olfactory receptor neurons. J Neurosci. 1992;12:915–23. [PMC free article: PMC6576063] [PubMed: 1312138]
- Bradley J., Bonigk W., Yau K.W., Frings S. Calmodulin permanently associates with rat olfactory CNG channels under native conditions. Nat Neurosci. 2004;7:705–10. [PMC free article: PMC2885912] [PubMed: 15195096]
- Bradley J., Reuter D., Frings S. Facilitation of calmodulin-mediated odor adaptation by cAMP-gated channel subunits. Science. 2001;294:2176–78. [PubMed: 11739960]
- Brunet L.J., Gold G.H., Ngai J. General anosmia caused by a targeted disruption of the mouse olfactory cyclic nucleotide-gated cation channel. Neuron. 1996;17:681–93. [PubMed: 8893025]
- Buck L., Axel R. A novel multigene family may encode odorant receptors: A molecular basis for odor recognition. Cell. 1991;65:175–87. [PubMed: 1840504]
- Caputo A., Caci E., Ferrera L., Pedemonte N., Barsanti C., Sondo E., Pfeffer U., Ravazzolo R., Zegarra-Moran O., Galietta L.J. TMEM16A, a membrane protein associated with calcium-dependent chloride channel activity. Science. 2008;322:590–94. [PubMed: 18772398]
- Castillo K., Delgado R., Bacigalupo J. Plasma membrane Ca(2+)-ATPase in the cilia of olfactory receptor neurons: Possible role in Ca(2+) clearance. Eur J Neurosci. 2007;26:2524–31. [PubMed: 17970729]
- Chen C., Nakamura T., Koutalos Y. Cyclic AMP diffusion coefficient in frog olfactory cilia. Biophys J. 1999;76:2861–67. [PMC free article: PMC1300257] [PubMed: 10233102]
- Chen T.Y., Takeuchi H., Kurahashi T. Odorant inhibition of the olfactory cyclic nucleotide-gated channel with a native molecular assembly. J Gen Physiol. 2006;128:365–71. [PMC free article: PMC2151561] [PubMed: 16940558]
- Chen T.Y., Yau K.W. Direct modulation by Ca(2+)-calmodulin of cyclic nucleotide-activated channel of rat olfactory receptor neurons. Nature. 1994;368:545–48. [PubMed: 7511217]
- Crumling M.A., Gold G.H. Ion concentrations in the mucus covering the olfactory epithelium in rodents. Soc Neurosci Abstr. 1998;24
- Dhallan R.S., Yau K.W., Schrader K.A., Reed R.R. Primary structure and functional expression of a cyclic nucleotide-activated channel from olfactory neurons. Nature. 1990;347:184–87. [PubMed: 1697649]
- Dzeja C., Hagen V., Kaupp U.B., Frings S. Ca2+ permeation in cyclic nucleotide-gated channels. EMBO J. 1999;18:131–44. [PMC free article: PMC1171109] [PubMed: 9878057]
- Firestein S., Picco C., Menini A. The relation between stimulus and response in olfactory receptor cells of the tiger salamander. J Physiol. 1993;468:1–10. [PMC free article: PMC1143811] [PubMed: 8254501]
- Firestein S., Shepherd G.M., Werblin F.S. Time course of the membrane current underlying sensory transduction in salamander olfactory receptor neurones. J Physiol. 1990;430:135–58. [PMC free article: PMC1181732] [PubMed: 2086763]
- Firestein S., Werblin F.S. Gated currents in isolated olfactory receptor neurons of the larval tiger salamander. Proc Natl Acad Sci USA. 1987;84:6292–96. [PMC free article: PMC299057] [PubMed: 2442756]
- Flannery R.J., French D.A., Kleene S.J. Clustering of cyclic-nucleotide-gated channels in olfactory cilia. Biophys J. 2006;91:179–88. [PMC free article: PMC1479050] [PubMed: 16603488]
- Frings S. Chloride-based signal amplification in olfactory sensory neurons. In: Alvarez-Leefmans F.J., Delpire E., editors. Physiology and Pathology of Chloride Transporters and Channels in the Nervous System. From Molecules to Diseases. New York: Academic Press; 2009. pp. 409–420.
- Frings S., Reuter D., Kleene S.J. Neuronal Ca2+-activated Cl– channels-homing in on an elusive channel species. Prog Neurobiol. 2000;60:247–89. [PubMed: 10658643]
- Getchell T.V. Functional properties of vertebrate olfactory receptor neurons. Physiol Rev. 1986;66:772–818. [PubMed: 3016769]
- Getchell T.V., Shepherd G.M. Adaptive properties of olfactory receptors analysed with odour pulses of varying durations. J Physiol. 1978;282:541–60. [PMC free article: PMC1282756] [PubMed: 722560]
- Grosmaitre X., Vassalli A., Mombaerts P., Shepherd G.M., Ma M. Odorant responses of olfactory sensory neurons expressing the odorant receptor MOR23: A patch clamp analysis in gene-targeted mice. Proc Natl Acad Sci USA. 2006;103:1970–75. [PMC free article: PMC1413638] [PubMed: 16446455]
- Grunwald M.E., Zhong H., Lai J., Yau K.W. Molecular determinants of the modulation of cyclic nucleotide-activated channels by calmodulin. Proc Natl Acad Sci USA. 1999;96:13444–49. [PMC free article: PMC23967] [PubMed: 10557340]
- Imanaka Y., Takeuchi H. Spiking properties of olfactory receptor cells in the slice preparation. Chem Senses. 2001;26:1023–27. [PubMed: 11595679]
- Jones D.T., Reed R.R. Golf: An olfactory neuron specific-G protein involved in odorant signal transduction. Science. 1989;244:790–95. [PubMed: 2499043]
- Jung A., Lischka F.W., Engel J., Schild D. Sodium/calcium exchanger in olfactory receptor neurones of Xenopus laevis. Neuroreport. 1994;5:1741–44. [PubMed: 7827321]
- Kaneko H., Nakamura T., Lindemann B. Noninvasive measurement of chloride concentration in rat olfactory receptor cells with use of a fluorescent dye. Am J Physiol Cell Physiol. 2001;280:C1387–C93. [PubMed: 11350733]
- Kaneko H., Putzier I., Frings S., Kaupp U.B., Gensch T. Chloride accumulation in mammalian olfactory sensory neurons. J Neurosci. 2004;24:7931–38. [PMC free article: PMC6729923] [PubMed: 15356206]
- Kerjaschki D. The central tubuli in distal segments of olfactory cilia lack dynein arms. Experientia. 1976;32:1459–60. [PubMed: 136362]
- Kleene S. Origin of the chloride current in olfactory transduction. Neuron. 1993;11:123–32. [PubMed: 8393322]
- Kleene S. Inhibition of olfactory cyclic nucleotide-activated current by calmodulin antagonists. Br J Pharmacol. 1994;111:469–72. [PMC free article: PMC1909980] [PubMed: 7516255]
- Kleene S. High-gain, low-noise amplification in olfactory transduction. Biophys J. 1997;73:1110–17. [PMC free article: PMC1181007] [PubMed: 9251827]
- Kleene S. Both external and internal calcium reduce the sensitivity of the olfactory cyclic-nucleotide-gated channel to cAMP. J Neurophysiol. 1999;81:2675–82. [PubMed: 10368387]
- Kleene S. The electrochemical basis of odor transduction in vertebrate olfactory cilia. Chem Senses. 2008;33:839–59. [PubMed: 18703537]
- Kleene S.J., Gesteland R.C. Calcium-activated chloride conductance in frog olfactory cilia. J Neurosci. 1991;11:3624–29. [PMC free article: PMC6575529] [PubMed: 1941099]
- Kleene S.J., Pun R.Y. Persistence of the olfactory receptor current in a wide variety of extracellular environments. J Neurophysiol. 1996;75:1386–91. [PubMed: 8727385]
- Kramer R.H., Siegelbaum S.A. Intracellular Ca2+ regulates the sensitivity of cyclic nucleotide-gated channels in olfactory receptor neurons. Neuron. 1992;9:897–906. [PubMed: 1384576]
- Kurahashi T. Activation by odorants of cation-selective conductance in the olfactory receptor cell isolated from the newt. J Physiol. 1989;419:177–92. [PMC free article: PMC1190003] [PubMed: 2621628]
- Kurahashi T. The response induced by intracellular cyclic AMP in isolated olfactory receptor cells of the newt. J Physiol. 1990;430:355–71. [PMC free article: PMC1181741] [PubMed: 1707967]
- Kurahashi T., Kaneko A. High density cAMP-gated channels at the ciliary membrane in the olfactory receptor cell. Neuroreport. 1991;2:5–8. [PubMed: 1722719]
- Kurahashi T., Kaneko A. Gating properties of the cAMP-gated channel in toad olfactory receptor cells. J Physiol. 1993;466:287–302. [PMC free article: PMC1175479] [PubMed: 8410695]
- Kurahashi T., Lowe G., Gold G.H. Suppression of odorant responses by odorants in olfactory receptor cells. Science. 1994;265:118–20. [PubMed: 8016645]
- Kurahashi T., Menini A. Mechanism of odorant adaptation in the olfactory receptor cell. Nature. 1997;385:725–29. [PubMed: 9034189]
- Kurahashi T., Shibuya T. Ca2(+)-dependent adaptive properties in the solitary olfactory receptor cell of the newt. Brain Res. 1990;515:261–68. [PubMed: 2113412]
- Kurahashi T., Yau K.W. Co-existence of cationic and chloride components in odorant-induced current of vertebrate olfactory receptor cells. Nature. 1993;363:71–74. [PubMed: 7683113]
- Lagostena L., Menini A. Whole-cell recordings and photolysis of caged compounds in olfactory sensory neurons isolated from the mouse. Chem Senses. 2003;28:705–16. [PubMed: 14627539]
- Lancet D. Vertebrate olfactory reception. Annu Rev Neurosci. 1986;9:329–55. [PubMed: 2423007]
- Larsson H.P., Kleene S.J., Lecar H. Noise analysis of ion channels in non-space-clamped cables: Estimates of channel parameters in olfactory cilia. Biophys J. 1997;72:1193–203. [PMC free article: PMC1184503] [PubMed: 9138566]
- Leinders Z., fall T., Rand M.N., Shepherd G.M., Greer C.A., Zufall F. Calcium entry through cyclic nucleotide-gated channels in individual cilia of olfactory receptor cells: Spatiotemporal dynamics. J Neurosci. 1997;17:4136–48. [PMC free article: PMC6573532] [PubMed: 9151731]
- Lidow M.S., Menco B.P. Observations on axonemes and membranes of olfactory and respiratory cilia in frogs and rats using tannic acid-supplemented fixation and photographic rotation. J Ultrastruct Res. 1984;86:18–30. [PubMed: 6204063]
- Liu M., Chen T.Y., Ahamed B., Li J., Yau K.W. Calcium-calmodulin modulation of the olfactory cyclic nucleotide-gated cation channel. Science. 1994;266:1348–54. [PubMed: 7526466]
- Lowe G., Gold G.H. The spatial distributions of odorant sensitivity and odorant-induced currents in salamander olfactory receptor cells. J Physiol. 1991;442:147–68. [PMC free article: PMC1179883] [PubMed: 1798028]
- Lowe G., Gold G.H. Nonlinear amplification by calcium-dependent chloride channels in olfactory receptor cells. Nature. 1993;366:283–86. [PubMed: 8232590]
- Lowe G., Nakamura T., Gold G.H. Adenylate cyclase mediates olfactory transduction for a wide variety of odorants. Proc Natl Acad Sci USA. 1989;86:5641–45. [PMC free article: PMC297680] [PubMed: 2787513]
- Lynch J.W., Barry P.H. Action potentials initiated by single channels opening in a small neuron (rat olfactory receptor). Biophys J. 1989;55:755–68. [PMC free article: PMC1330559] [PubMed: 2470428]
- Lynch J.W., Lindemann B. Cyclic nucleotide-gated channels of rat olfactory receptor cells: Divalent cations control the sensitivity to cAMP. J Gen Physiol. 1994;103:87–106. [PMC free article: PMC2216850] [PubMed: 7513349]
- Ma M., Chen W.R., Shepherd G.M. Electrophysiological characterization of rat and mouse olfactory receptor neurons from an intact epithelial preparation. J Neurosci Methods. 1999;92:31–40. [PubMed: 10595701]
- Ma M., Grosmaitre X., Iwema C.L., Baker H., Greer C.A., Shepherd G.M. Olfactory signal transduction in the mouse septal organ. J Neurosci. 2003;23:317–24. [PMC free article: PMC2227318] [PubMed: 12514230]
- Matsuzaki O., Bakin R.E., Cai X., Menco B.P., Ronnett G.V. Localization of the olfactory cyclic nucleotide-gated channel subunit 1 in normal, embryonic and regenerating olfactory epithelium. Neuroscience. 1999;94:131–40. [PubMed: 10613503]
- Matthews H.R., Reisert J. Calcium, the two-faced messenger of olfactory transduction and adaptation. Curr Opin Neurobiol. 2003;13:469–75. [PubMed: 12965295]
- Mayer U., Kuller A., Daiber P.C., Neudorf I., Warnken U., Schnolzer M., Frings S., Mohrlen F. The proteome of rat olfactory sensory cilia. Proteomics. 2009;9:322–34. [PubMed: 19086097]
- Menco B.P. Qualitative and quantitative freeze-fracture studies on olfactory and nasal respiratory structures of frog, ox, rat, and dog. I. A general survey. Cell Tissue Res. 1980;207:183–209. [PubMed: 6966972]
- Menco B.P. Ultrastructural aspects of olfactory signaling. Chem Senses. 1997;22:295–311. [PubMed: 9218142]
- Menco B.P., Morrison E.E. Morphology of the mammalian olfactory epithelium: Form, fine structure, function, and pathology. In: Doty R., editor. Handbook of Olfaction and Gustation. New York: Marcel Dekker; 2003. pp. 17–49.
- Menini A. Cyclic nucleotide-gated channels in visual and olfactory transduction. Biophys Chem. 1995;55:185–96. [PubMed: 7542935]
- Menini A. Calcium signalling and regulation in olfactory neurons. Curr Opin Neurobiol. 1999;9:419–26. [PubMed: 10448159]
- Michalakis S., Reisert J., Geiger H., Wetzel C., Zong X., Bradley J., Spehr M., Huttl S., Gerstner A., Pfeifer A., Hatt H., Yau K.W., Biel M. Loss of CNGB1 protein leads to olfactory dysfunction and subciliary cyclic nucleotide-gated channel trapping. J Biol Chem. 2006;281:35156–66. [PMC free article: PMC2885922] [PubMed: 16980309]
- Mombaerts P. Genes and ligands for odorant, vomeronasal and taste receptors. Nat Rev Neurosci. 2004;5:263–78. [PubMed: 15034552]
- Munger S.D., Lane A.P., Zhong H., Leinders Z., fall T., Yau K.W., Zufall F., Reed R.R. Central role of the CNGA4 channel subunit in Ca2+-calmodulin-dependent odor adaptation. Science. 2001;294:2172–75. [PMC free article: PMC2885906] [PubMed: 11739959]
- Nache V., Schulz E., Zimmer T., Kusch J., Biskup C., Koopmann R., Hagen V., Benndorf K. Activation of olfactory-type cyclic nucleotide-gated channels is highly cooperative. J Physiol. 2005;569:91–102. [PMC free article: PMC1464204] [PubMed: 16081488]
- Nakamura T., Gold G.H. A cyclic nucleotide-gated conductance in olfactory receptor cilia. Nature. 1987;325:442–44. [PubMed: 3027574]
- Nickell W.T., Kleene N.K., Gesteland R.C., Kleene S.J. Neuronal chloride accumulation in olfactory epithelium of mice lacking NKCC1. J Neurophysiol. 2006;95:2003–6. [PMC free article: PMC1379662] [PubMed: 16319203]
- Nickell W.T., Kleene N.K., Kleene S.J. Mechanisms of neuronal chloride accumulation in intact mouse olfactory epithelium. J Physiol. 2007;583:1005–20. [PMC free article: PMC2277205] [PubMed: 17656441]
- Noe J., Tareilus E., Boekhoff I., Breer H. Sodium/calcium exchanger in rat olfactory neurons. Neurochem Int. 1997;30:523–31. [PubMed: 9152993]
- Ottoson D. Analysis of the electrical activity of the olfactory epithelium. Acta Physiol Scand Suppl. 1955;35:1–83. [PubMed: 13301864]
- Pifferi S., Boccaccio A., Menini A. Cyclic nucleotide-gated ion channels in sensory transduction. FEBS Lett. 2006a;580:2853–59. [PubMed: 16631748]
- Pifferi S., Dibattista M., Menini A. TMEM16B induces chloride currents activated by calcium in mammalian cells. Pflugers Arch. 2009 doi: 10.1007/s00424-009-0684-9. [PubMed: 19475416]
- Pifferi S., Pascarella G., Boccaccio A., Mazzatenta A., Gustincich S., Menini A., Zucchelli S. Bestrophin-2 is a candidate calcium-activated chloride channel involved in olfactory transduction. Proc Natl Acad Sci USA. 2006b;103:12929–34. [PMC free article: PMC1568948] [PubMed: 16912113]
- Pyrski M., Koo J.H., Polumuri S.K., Ruknudin A.M., Margolis J.W., Schulze D.H., Margolis F.L. Sodium/calcium exchanger expression in the mouse and rat olfactory systems. J Comp Neurol. 2007;501:944–58. [PubMed: 17311327]
- Reisert J., Bauer P.J., Yau K.W., Frings S. The Ca-activated Cl channel and its control in rat olfactory receptor neurons. J Gen Physiol. 2003;122:349–63. [PMC free article: PMC2234486] [PubMed: 12939394]
- Reisert J., Lai J., Yau K.W., Bradley J. Mechanism of the excitatory Cl– response in mouse olfactory receptor neurons. Neuron. 2005;45:553–61. [PMC free article: PMC2877386] [PubMed: 15721241]
- Reisert J., Matthews H.R. Na+-dependent Ca2+ extrusion governs response recovery in frog olfactory receptor cells. J Gen Physiol. 1998;112:529–35. [PMC free article: PMC2229439] [PubMed: 9806962]
- Reisert J., Matthews H.R. Adaptation of the odour-induced response in frog olfactory receptor cells. J Physiol. 1999;519(3):801–13. [PMC free article: PMC2269541] [PubMed: 10457092]
- Reisert J., Matthews H.R. Response properties of isolated mouse olfactory receptor cells. J Physiol. 2001;530:113–22. [PMC free article: PMC2278387] [PubMed: 11136863]
- Reuter D., Zierold K., Schroder W.H., Frings S. A depolarizing chloride current contributes to chemoelectrical transduction in olfactory sensory neurons in situ. J Neurosci. 1998;18:6623–30. [PMC free article: PMC6792953] [PubMed: 9712634]
- Schild D., Restrepo D. Transduction mechanisms in vertebrate olfactory receptor cells. Physiol Rev. 1998;78:429–66. [PubMed: 9562035]
- Schroeder B.C., Cheng T., Jan Y.N., Jan L.Y. Expression cloning of TMEM16A as a calcium-activated chloride channel subunit. Cell. 2008;134:1019–29. [PMC free article: PMC2651354] [PubMed: 18805094]
- Scott J.W., Scott-Johnson P.E. The electroolfactogram: A review of its history and uses. Microsc Res Tech. 2002;58:152–60. [PubMed: 12203693]
- Song Y., Cygnar K.D., Sagdullaev B., Valley M., Hirsh S., Stephan A., Reisert J., Zhao H. Olfactory CNG channel desensitization by Ca2+/CaM via the B1b subunit affects response termination but not sensitivity to recurring stimulation. Neuron. 2008;58:374–86. [PMC free article: PMC2587172] [PubMed: 18466748]
- Stephan A.B., Shum E.Y., Hirsh S., Cygnar K.D., Reisert J., Zhao H. ANO2 is the cilial calcium-activated chloride channel that may mediate olfactory amplification. Proc Natl Acad Sci U S A. 2009 doi: 10.1073/pnas.0903304106. [PMC free article: PMC2702256] [PubMed: 19561302]
- Takeuchi H., Imanaka Y., Hirono J., Kurahashi T. Cross-adaptation between olfactory responses induced by two subgroups of odorant molecules. J Gen Physiol. 2003;122:255–64. [PMC free article: PMC2234484] [PubMed: 12939391]
- Takeuchi H., Ishida H., Hikichi S., Kurahashi T. Mechanism of olfactory masking in the sensory cilia. J Gen Physiol. 2009;133:583–601. [PMC free article: PMC2713142] [PubMed: 19433623]
- Takeuchi H., Kurahashi T. Photolysis of caged cyclic AMP in the ciliary cytoplasm of the newt olfactory receptor cell. J Physiol. 2002;541:825–33. [PMC free article: PMC2290348] [PubMed: 12068043]
- Takeuchi H., Kurahashi T. Identification of second messenger mediating signal transduction in the olfactory receptor cell. J Gen Physiol. 2003;122:557–67. [PMC free article: PMC2229575] [PubMed: 14581582]
- Takeuchi H., Kurahashi T. Mechanism of signal amplification in the olfactory sensory cilia. J Neurosci. 2005;25:11084–91. [PMC free article: PMC6725648] [PubMed: 16319308]
- Takeuchi H., Kurahashi T. Distribution, amplification, and summation of cyclic nucleotide sensitivities within single olfactory sensory cilia. J Neurosci. 2008;28:766–75. [PMC free article: PMC6670341] [PubMed: 18199776]
- Tirindelli R., Dibattista M., Pifferi S., Menini A. From pheromones to behavior. Physiol Rev. 2009;89:921–56. [PubMed: 19584317]
- Tomaru A., Kurahashi T. Mechanisms determining the dynamic range of the bullfrog olfactory receptor cell. J Neurophysiol. 2005;93:1880–88. [PubMed: 15548631]
- Torre V., Ashmore J.F., Lamb T.D., Menini A. Transduction and adaptation in sensory receptor cells. J Neurosci. 1995;15:7757–68. [PMC free article: PMC6577959] [PubMed: 8613717]
- Uebi T., Miwa N., Kawamura S. Comprehensive interaction of dicalcin with annexins in frog olfactory and respiratory cilia. FEBS J. 2007;274:4863–76. [PubMed: 17714509]
- Usukura J., Yamada E. Observations on the cytolemma of the olfactory receptor cell in the newt. 1. Freeze replica analysis. Cell Tissue Res. 1978;188:83–98. [PubMed: 639099]
- Varnum M.D., Zagotta W.N. Interdomain interactions underlying activation of cyclic nucleotide-gated channels. Science. 1997;278:110–13. [PubMed: 9311913]
- Weeraratne S.D., Valentine M., Cusick M., Delay R., Van Houten J.L. Plasma membrane calcium pumps in mouse olfactory sensory neurons. Chem Senses. 2006;31:725–30. [PMC free article: PMC2442823] [PubMed: 16855061]
- Yan C., Zhao A.Z., Bentley J.K., Loughney K., Ferguson K., Beavo J.A. Molecular cloning and characterization of a calmodulin-dependent phosphodiesterase enriched in olfactory sensory neurons. Proc Natl Acad Sci USA. 1995;92:9677–81. [PMC free article: PMC40865] [PubMed: 7568196]
- Yang Y.D., Cho H., Koo J.Y., Tak M.H., Cho Y., Shim W.S., Park S.P., Lee J., Lee B., Kim B.M., Raouf R., Shin Y.K., Oh U. TMEM16A confers receptor-activated calcium-dependent chloride conductance. Nature. 2008;455:1210–15. [PubMed: 18724360]
- Yu T.T., McIntyre J.C., Bose S.C., Hardin D., Owen M.C., McClintock T.S. Differentially expressed transcripts from phenotypically identified olfactory sensory neurons. J Comp Neurol. 2005;483:251–62. [PMC free article: PMC2967457] [PubMed: 15682396]
- Zheng J., Varnum M.D., Zagotta W.N. Disruption of an intersubunit interaction underlies Ca2+-calmodulin modulation of cyclic nucleotide-gated channels. J Neurosci. 2003;23:8167–75. [PMC free article: PMC6740483] [PubMed: 12954880]
- Zheng J., Zagotta W.N. Stoichiometry and assembly of olfactory cyclic nucleotide-gated channels. Neuron. 2004;42:411–21. [PubMed: 15134638]
- Zippel H.P. Historical aspects of research on the vertebrate olfactory system. Naturwissenschaften. 1993;80:65–76. [PubMed: 8446176]
- Zufall F., Firestein S. Divalent cations block the cyclic nucleotide-gated channel of olfactory receptor neurons. J Neurophysiol. 1993;69:1758–68. [PubMed: 7685377]
- Zufall F., Shepherd G.M., Firestein S. Inhibition of the olfactory cyclic nucleotide gated ion channel by intracellular calcium. Proc R Soc Lond B Biol Sci. 1991;246:225–30. [PubMed: 1686087]
- Review Engineering Aspects of Olfaction.[Neuromorphic Olfaction. 2013]Review Engineering Aspects of Olfaction.Persaud KC. Neuromorphic Olfaction. 2013
- The Ca(2+)-activated Cl(-) channel TMEM16B shapes the response time course of olfactory sensory neurons.[J Physiol. 2024]The Ca(2+)-activated Cl(-) channel TMEM16B shapes the response time course of olfactory sensory neurons.Reisert J, Pifferi S, Guarneri G, Ricci C, Menini A, Dibattista M. J Physiol. 2024 Oct; 602(19):4889-4905. Epub 2024 Aug 21.
- Ca2+-activated Cl- channels of the ClCa family express in the cilia of a subset of rat olfactory sensory neurons.[PLoS One. 2013]Ca2+-activated Cl- channels of the ClCa family express in the cilia of a subset of rat olfactory sensory neurons.Gonzalez-Silva C, Vera J, Bono MR, González-Billault C, Baxter B, Hansen A, Lopez R, Gibson EA, Restrepo D, Bacigalupo J. PLoS One. 2013; 8(7):e69295. Epub 2013 Jul 9.
- Single Ca(2+)-activated Cl(-) channel currents recorded from toad olfactory cilia.[BMC Neurosci. 2016]Single Ca(2+)-activated Cl(-) channel currents recorded from toad olfactory cilia.Delgado R, Mura CV, Bacigalupo J. BMC Neurosci. 2016 Apr 25; 17(1):17. Epub 2016 Apr 25.
- Review Anoctamin 2/TMEM16B: a calcium-activated chloride channel in olfactory transduction.[Exp Physiol. 2012]Review Anoctamin 2/TMEM16B: a calcium-activated chloride channel in olfactory transduction.Pifferi S, Cenedese V, Menini A. Exp Physiol. 2012 Feb; 97(2):193-9. Epub 2011 Sep 2.
- Signal Transduction in Vertebrate Olfactory Cilia - The Neurobiology of Olfactio...Signal Transduction in Vertebrate Olfactory Cilia - The Neurobiology of Olfaction
Your browsing activity is empty.
Activity recording is turned off.
See more...